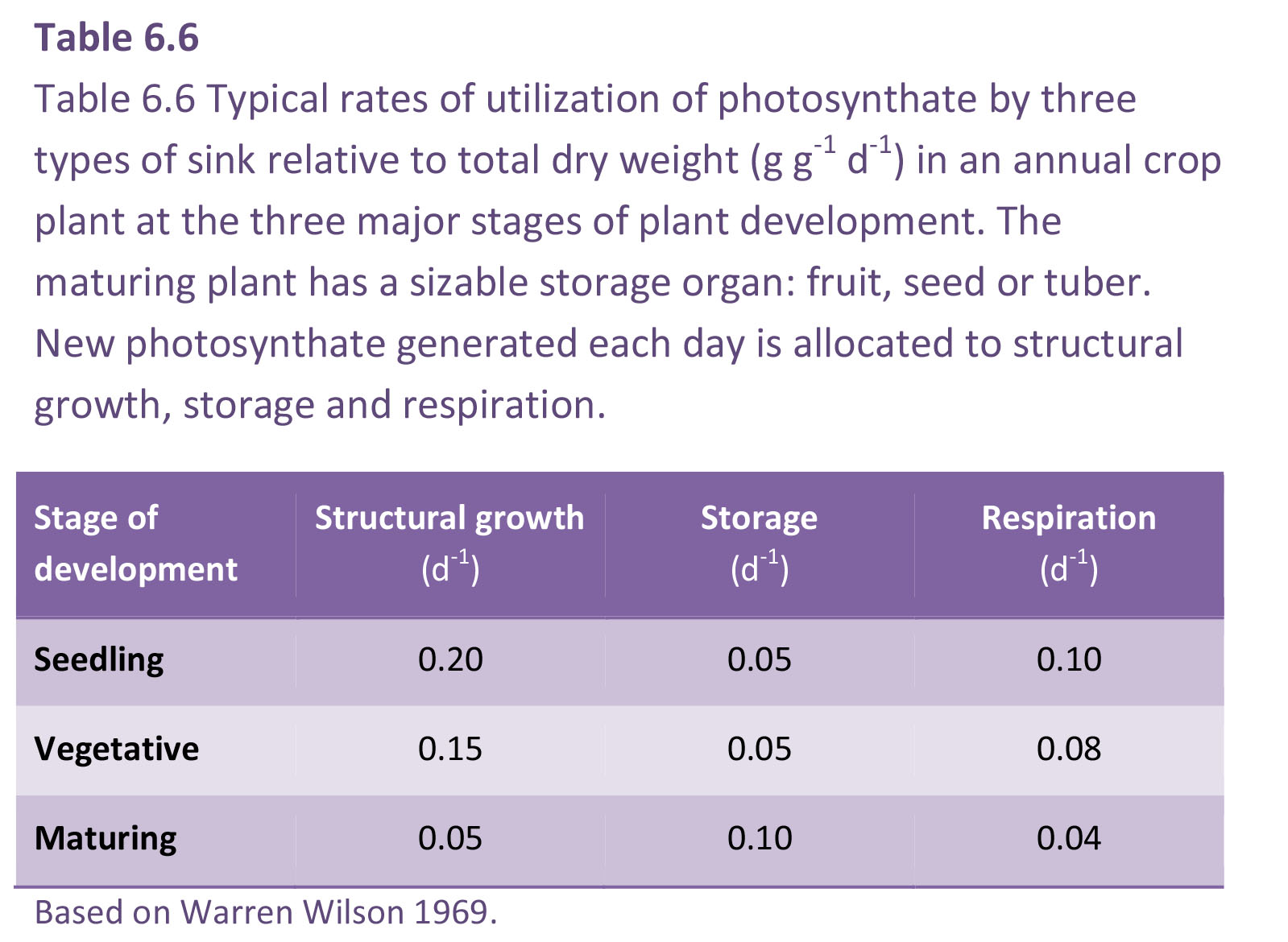
A significant amount of the CO2 fixed by photosynthesis is respired to produce the energy needed for production of new organs and maintenance of old ones. This is often termed a “cost”.
Costs associated with growth and maintenance of vascular plants can be represented as biomass equivalents. Calculations for dry matter utilisation during growth and development (Table 6.6) show that respiratory loss is substantial and can range from about 20-40% of the dry matter produced.
During growth and development (Table 6.6) a fall in structural growth rate has been accompanied by a fall in whole-plant respiration, while the amount of photosynthate allocated to storage has risen. Overall, respiration accounts for a significant fraction of photoassimilate. Commonly one-third and, under stressful conditions as much as two-thirds, of a plant’s daily fixed CO2 can be respired.
According to the estimates in Table 6.6, a germinating seedling with starting biomass of 1 g has in one day gained a further 0.2 g in structural growth plus 0.05 g in storage, with respiratory costs equivalent to 0.10 g g–1 d–1, or 40% of the dry matter formed. Using similar logic, the young vegetative plant has produced structural growth and storage at a respiratory cost equivalent to 0.08 g g–1 d–1, also 40% of the dry matter formed. In a maturing plant with less structural growth and with storage organs that are importing photoassimilate, the respiratory cost has fallen to 0.04 g g–1 d–1 or 27%, as the production of storage compounds requires less energy than does structural growth.
The physiological and biochemical processes involved in energy production, respiration, and utilization of energy have been described in detail in Chapter 2, but for convenience a summary is presented in the next section.
Figure 6.17. Simplified view of processes involved in carbon gain and generation of respiratory energy. The figure represents a mesophyll cell in a leaf. CO2 assimilated by chloroplasts produces carbon-rich compounds (photoassimilates) that are exported to the cytosol and mitochondria. CO2 is then produced during breakdown of these carbon-rich compounds by glycolysis and by mitochondrial respiration. Release of CO2 and uptake of O2 by mitochondria are coupled to production of usable energy (ATP, NADH). Carbon skeletons (necessary for protein synthesis) are also produced during mitochondrial respiration (Original drawing courtesy Owen Atkin)
Photoassimilate is used to generate respiratory products needed for plant growth (Figure 6.17). Carbohydrate compounds produced from photosynthesis are exported from chloroplasts to the cytosol and mitochondria, and used to generate ATP, redox equivalents (in particular NADH) and carbon skeletons via glycolysis, mitochondrial tricarboxylic acid (TCA) activity and mitochondrial electron transport. Generation of these respiratory products results in CO2 loss during glycolysis and passage of metabolites around the TCA cycle.
Energy (ATP and NADH) and carbon skeletons produced by mitochondrial respiration are used for various processes essential to growth, maintenance, nutrient uptake and transport within the plant.
Maintenance respiration represents the portion of respiratory CO2 release that is coupled to production of energy (ATP and reducing power) necessary for maintenance of chemical and electrochemical gradients across membranes, turnover of cellular constituents such as proteins, and processes involved in physiological acclimation to changing or harsh environments (Penning de Vries 1975). Energy needed for maintenance is determined by the specific costs of processes taking place and is generally regarded as proportional to tissue mass.
Protein turnover is an energy-intensive process accounting for 60–80% of maintenance respiration (Penning de Vries 1975). Demand for respiratory energy associated with protein turnover will depend on turnover rate, respiratory costs associated with turnover, as well as the total amount of proteins undergoing turnover. Enzymes such as nitrate reductase (a key enzyme involved in nitrogen assimilation) have a very high turnover rate (Amthor 1984). As a result, plants assimilating nitrate have higher maintenance requirements than ammonium-grown plants (Hansen 1979).
Translocation of photoassimilate is also a potentially expensive process that accounts for approximately 30% of total dark respiration in several starch-storing plant species and would represent a substantial drain on photo-assimilate that could otherwise go into storage organs (Table 6.6). Phloem loading and unloading is largely responsible for this high cost because transport of sugars between symplasm and apoplasm depends on cotransport of H+. Movement of H+ is in turn dependent on ATP being consumed in the symplasm (Chapter 5). Traffic in photoassimilate thus increases demand for maintenance respiration
Energy costs associated with nutrient aquisition are often very high because ions have to be transported across root cell membranes using active transport systems that require substantial amounts of ATP. Energy requirement for ion uptake will depend on several factors, including the degree to which absorbed nutrients are released back to the soil and the degree to which protons and anions are cotransported into roots.
Growth respiration covers synthesis of new biomass from photosynthate and mineral nutrients and is regarded as proportional to the rate at which new material is being formed. Specific respiratory costs associated with growth (i.e. construction cost) will depend to a large extent on the chemical composition of plant material and by implication the amount of energy embedded in these molecules (Table 6.7). Compounds with a high carbon concentration require more ATP and reducing power for their synthesis (Lambers and Poorter 1992). For example, biomass stored as lipid represents an investment of almost three times as much energy as would be required for storage of the same mass of non-structural carbohydrate. Plant growth analysis based on dry mass accumulation takes no account of such differences in chemical composition of end-products, so that comparisons of growth efficiencies based solely on RGR of biomass must be viewed circumspectly.
Construction cost, and thus growth respiration, also varies according to the chemical form of available nitrogen (e.g. N2, NO3– and/or NH4+) and sites of assimilation. Nitrogen reduction is an energetically expensive process, requiring considerable input of respiratory energy (e.g. ATP + reductant) and TCA cycle intermediates. Plants fixing atmospheric N2 in their roots demand much ATP, namely 12.5–26.5 mol ATP per mol of NH4+ produced, and a further 2.5–3.0 mol ATP for subsequent assimilation into nitrogen-based metabolites such as amino acids and proteins. NO3– reduction to NH4+ is cheaper, costing around 12 mol ATP per mol NH4+ produced.
Respiratory costs associated with NO3– assimilation can be substantially reduced if reduction of NO3– to NH4+ and subsequent assimilation of NH4+ into amino acids takes place in leaves. Reduction and assimilation of NO3– can then used excess photosynthetic reductant and ATP. Growth respiration associated with synthesis of nitrogen-based resources is thus greatly reduced by shoot assimilation of NO3–.
Plants vary in their intrinsic growth rate, and may under the same environment differ three-fold in the relative growth rate. Some species are inherently fast-growing, and some inherently slow-growing. This section investigates the reason for this, and in particular the energy efficiency of the various species.
Fast-growing species tend to have higher rates of photosynthesis, but also use respiratory energy more efficiently for maintenance, growth and ion uptake. Variations in efficiency of energy use reflect differences in the proportion of whole-plant respiration that is allocated to these three processes and/or the specific costs of each process.
Fast-growing species achieve a higher RGR under optimum conditions than do slow-growing species under similar conditions. Carbon loss via respiration is considerable with genetic differences in generation and utilisation of respiratory energy contributing to these differences in RGR. Fast-growing species achieve a higher RGR than slow-growing species because their net rate of CO2 uptake per unit of shoot and whole-plant mass is greater (Figure 6.18). By definition, net carbon fixed per day must depend to some extent on the proportion of fixed CO2 that is subsequently lost by respiration, so that differences in respiratory CO2 loss have an important impact on net carbon gain, and can be linked quantitatively to RGR.
Figure 6.18. Daily carbon economy of plant species that differ with respect to inherent maximum RGR (g g-1 d-1). The fast-growing grass and fast growing herb both exhibit higher rates of gross photosynthetic CO2 uptake per unit mass (i.e. net photosynthesis plus shoot dark respiration) than their slow-growing counterparts. Fast-growing species lose a smaller percentage of daily fixed carbon via respiration. Based on data in Atkin et al. (1996) Funct Ecol 10, 698-707 for slow-growing Australian alpine and fast-growing lowland Poa species, and Poorter et al. (1990) Plant Physiol 94, 621-627 for the slow-growing herb Pimpinella saxifrage versus the fast-growing herb Galinsoga parviflora).
Data shown in Figure 6.18 can be used to calculate RGR for each species from photosynthesis and respiration measurements if the plant’s carbon concentration is known.
Processes supporting a net gain in new biomass (dW, g) per unit time (dt, d) can be represented as:
\[\frac{\text{d}W}{\text{d}t}=A-R\tag{6.25}\]
where A is daily carbon assimilation and R is whole-plant respiratory loss, so that net gain per unit existing plant biomass per unit time (or RGR, g g–1 d–1) becomes
\[\text{RGR} = \frac{1}{W}\frac{\text{d}W}{\text{d}t} = \frac{A}{W} - \frac{R}{W} \tag{6.26}\]
If A and R are expressed as mmol carbon g–1 dry matter per day, then Equation 6.26 becomes
\[\text{RGR} = (A-R)/C_{wp} \tag{6.27}\]
where Cwp is plant carbon concentration in mmol carbon g–1 dry matter. R can be separated into Rshoot and Rroot.
Whole-plant RGR can now be linked to gas exchange data for shoot assimilation (A), shoot respiration (Rshoot) and root respiration (Rroot) according to the expression
\[\text{RGR} = (A-(R_{\text{shoot}} + R_{\text{root}})/C_{wp} \tag{6.28}\]
A and R can be determined from direct measurement of whole-plant gas exchange. The example below uses a value for Cwp of 34.8 mmol C g–1 dry matter.
Taking the fast-growing grass in Figure 6.18, where A is 11.1 mmol C g-1 plant d-1, and R for shoots and roots is 1.75 and 1.68 mmol C g-1 d-1 , then RGR = 0.22 g g–1 d–1 .
This prediction of 0.22 d–1 for RGR represents an instantaneous value derived from whole-plant gas exchange measurements, whereas 0.255 d–1 in Figure 6.18 represents an average RGR from growth analysis over several days. Gas exchange values are generally within 10% of RGR values from sequential harvests.
Herbs and grasses can differ in the degree to which respiratory losses account for differences in RGR. Considering grasses (Figure 6.18, left side), 56% of daily fixed CO2 is lost by respiration in the slow-growing alpine species whereas only 30% of daily fixed CO2 is respired by the fast-growing lowland grass species. Over half of the carbon loss is attributable to roots in both species and, overall, respiration rate per unit plant mass is slightly higher in the slow-growing grass species.
Herbs in Figure 6.18 (right side) differ from grasses because the fast-growing herb respires faster than the slow-growing herb (on a mass basis) so that differences in percentage loss of carbon between these species cannot be due to differences in respiration rates per se. Significantly, however, the fast-growing herb still loses a smaller percentage of daily fixed carbon due to whole-plant respiration because daily CO2 assimilation (mass basis) is especially high. A notably higher SLA in this fast-growing herb contributes to faster photo-synthesis on a mass basis (Figure 6.18).
A lower percentage loss of daily fixed carbon due to respiration in fast-growing grasses and fast-growing herbs does imply that carbon metabolism is more effective in these species than in their slow-growing counterparts, and serves as a model for generalisations. Such fast-growing plants may be more efficient in how they generate and/or use respiratory energy.
It is likely that an inherent capacity for fast growth confers a selective advantage for plants in favourable environments such as warm moist lowlands, but would be selectively neutral in restrictive environments such as nutritionally poor sites or alpine regions.
Growth respiration can be distinguished from maintenance respiration by relating variation in respiration rate to variation in RGR over short time intervals (Figure 6.19; Penning de Vries 1975). This approach assumes a model for respiration where:
\[\text{Total respiration} = \text{Maintenance respiration} + \text{(Specific costs of growth} \times \text {RGR)} \tag{6.31}\]
where respiration is expressed in mmol CO2 g-1 d-1, and specific costs of growth in mmol CO2 g-1.
Figure 6.19. Determination of growth and maintenance respiration in whole plants, roots or shoots. Respiration rates are plotted as a function of RGR and maintenance respiration is taken as the rate of respiration when RGR is extrapolated to zero. The slope of this plot (25 mmol CO2 g-1) provides an estimate of the specific costs of growth which are assumed to remain constant for a given plant regardless of RGR. Variation in both RGR and respiration rate can be generated in several ways, including growing plants under different irradiances, or by measuring respiration and growth rates during development (RGR and respiration rate commonly decrease with age) (Original drawing courtesy Owen Atkin)
Decreases in RGR (e.g. due to growth under different irradiance or during ageing) are assumed to decrease demand for growth respiration, whereas demand for maintenance respiration is assumed to remain constant at different RGR values. Based on these assumptions, the maintenance component can be estimated by extrapolating the respiration rate back to a point where no growth occurs (1 mmol CO2 g–1 d–1 in Figure 6.19). Specific respiratory costs associated with growth can be estimated from the slope of the respiration–RGR plot (25 mmol CO2 g–1 in Figure 6.19).
An alternative approach to maintenance and growth components of respiration involves holding plants in extended darkness. Most annual plants use up their readily available energy sources after about 2 d and shoot growth will cease. Rate of CO2 release would then reflect the maintenance component of dark respiration. The difference in dark respiration rates before and after 2 d darkness would be the growth component.
Such methods incorporate specific costs of ion uptake into estimates of growth respiration, but do not isolate the ion uptake component of root respiration. Ion uptake respiration can be separated from growth by partitioning root respiration into growth, maintenance and ion uptake components. The approach adopted by Veen (1980) assumes a model where:
\[\text{Root respiration} = \text{Maintenance respiration} + \text{(Specific costs of growth} \times \text {RGR)} + \text{(Specific costs of ion uptake} \times \text{Ion uptake rate)} \tag{6.31}\]
A multiple regression analysis approach can be used to separate these components (Figure 6.20). Root respiration is taken as a dependent variable; while RGR and ion uptake rate are independent variables (van der Werf et al. 1994). The maintenance component of root respiration is taken as the rate of respiration when growth and ion uptake are extrapolated back to zero. Specific costs of growth and ion uptake are taken as the slope of the respiration versus growth and ion uptake regressions, respectively.
Figure 6.20. Determination of growth, maintenance and ion uptake components of root respiration. Maintenance respiration is taken as the rate of respiration when ion uptake rate and relative growth rate (RGR) are extrapolated to zero. Specific costs of ion uptake are estimated from the slope of the respiration versus ion uptake rate plot, while the actual amount of respiration allocated to ion uptake is shown. The slope of respiration versus RGR represents the specific costs of growth. Growth respiration varies with RGR, but specific costs of growth, ion uptake and maintenance are assumed to remain constant irrespective of variation in RGR or ion uptake (Original drawing courtesy Owen Atkin)
Most respiratory energy is allocated to nutrient acquisition in both fast- and slow-growing species (Figure 6.21) and this proportion increases even further under suboptimal conditions as maintenance costs rise. However, fast-growing species are distinguished by allocating less respiratory energy to nutrient acquisition, and more to growth. Presumably, a lower allocation to ion uptake in fast-growing species arises from lower specific costs. Loss of absorbed nutrients could also be lower in fast-growing species, while cotransport of protons and anions into roots might conserve energy. Maintenance costs also appear to be slightly lower in fast-growing plants (Figure 6.21) but any difference between these two plant categories in allocation to maintenance processes is small and is unlikely to matter overall. Nevertheless, differences in maintenance respiration will become more important when a plant is exposed to unfavourable conditions which invariably increase allocation of respiratory energy to fine-root turnover and maintenance of those structures.
Nitrogen limitation decreases absolute rates of shoot and root respiration in both fast- and slow-growing species (Figure 6.22) but the decrease in gross photosynthesis is much greater. Thus, the percentage of daily fixed CO2 lost during respiration increases under nitrogen limitation. This mainly results from a greater allocation of photoassimilate to roots. Slower growth of whole plants on low nitrogen is therefore due to both slower photosynthesis due to less Rubisco coupled with more costly nitrogen acquisition.
Figure 6.22. Low nitrogen (supplied as nitrate) reduces RGR in both fast-growing and slow-growing grass species. Photosynthesis and respiration (mass basis) also decrease, but the percentage of daily fixed carbon that is lost via respiration is higher on low nitrogen due to a greater investment of photoassimilate in roots. Photosynthetic CO2 gain is expressed as net photosynthesis plus shoot respiration (assuming shoots respire in daytime at the same rate as that measured in darkness). Values for CO2 exchange per unit plant mass were calculated from whole-plant measurements and proportions of plant biomass allocated to shoots and root, respectively. Based on Poorter et al. (1995) Plant Soil 171, 217-227
The proportion of daily fixed CO2 that is respired may also increase under other stressful conditions such as drought, high temperature and ion toxicity. Challenged by such stresses, a greater proportion of respiratory energy is being used to support cellular maintenance in place of growth.
In conclusion, this chapter has shown that respiratory costs are high, for both formation of new tissues and maintenance of old ones. Plants profit from shedding old leaves and roots, where the costs of maintenance outweigh the benefits of their function. Future research into ways to minimise costs while maximising functions may produce more efficient plant forms. Quantitative growth analyses will be essential in developing new plants or improving management practises for higher yields in both optimal and suboptimal environments.