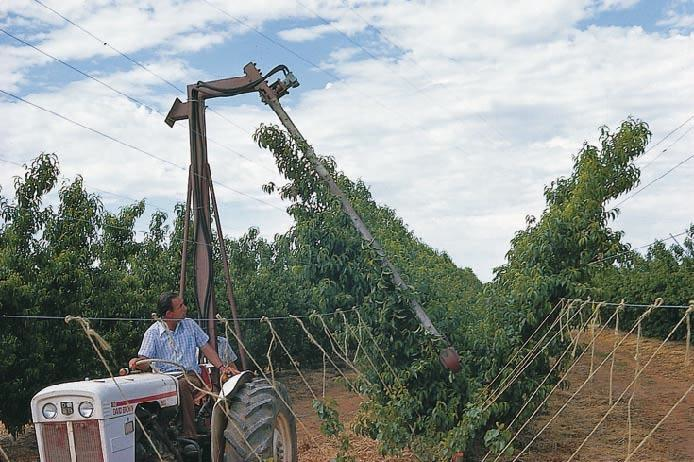
Figure 12.27 Peach trees trained to a Tatura trellis. (Photograph courtesy P.E. Kriedemann)
An upper limit on dry matter production by crops is set by the amount of visible radiation (400–700 nm) intercepted (Monteith 1977). Horticultural crops, however, rarely achieve 100% interception of sunlight because physical access is required year round for routine management operations such as spraying, pruning and picking. This is especially so for perennial fruit crops and vines, but is also the case for many annual greenhouse and vegetable crops that are repeatedly hand harvested. With a closed agronomic canopy such as a field of wheat, light interception is largely determined by LAI. With discontinuous canopies such as an apple orchard or a vineyard, a fixed LAI can intercept different amounts of light depending upon the display in space of the discrete canopy units, namely individual trees or vines. (LAI for discontinuous canopies is calculated from the total area of leaves on the plant divided by the area allocated to the plant.)
Light interception of horticultural crops can be altered by changing spacing, tree height, tree shape and/or row orientation. An illustration of this is given in Table 12.1 using a simulation model of light interception (Palmer 1989). The model calculated light interception over a whole day and allowed for change in incident radiation, sun angle (both elevation and azimuth) and sky light. The model was used to calculate light interception under sunny conditions by apple trees arranged in continuous rows, of truncated conical cross-section, in midsummer at Riwaka, Nelson, New Zealand. For a constant LAI, light interception increased by increasing hedge height, closer row spacings and planting in a north–south rather than an east–west orientation (Table 12.1). Light interception varied by a factor of two, from 27% by short wide-spaced hedges in east–west (EW) rows to 55% by tall close spacings in north–south (NS) rows. Differences due to row orientation will be smaller in cloudy regions where more of the incoming light is diffuse.
In Table 12.1, the density of leaves within the tree volume changes with height and row spacing to maintain a constant LAI. Differences in light interception would be much larger if leaf area density rather than LAI was maintained at a constant. Nevertheless, data given in Table 12.1 for discontinuous canopies show that the relationship between LAI and light interception depends on the physical arrangement of foliage in space. Calculations for Table 12.1 assume an extinction coefficient appropriate for apples. Light interception would have to be recalculated for other crops such as peach and cherry that have lower extinction coefficients (Flore 1994).
As discussed in Section 12.3, the relationship between light interception (\(F\)) and LAI of a continuous canopy follows the Beer–Lambert Law, and can be approximated by Equation 12.5. Again, \(k\) (the extinction coefficient) is dependent upon leaf angle distribution, leaf transmission to sunlight and whether the foliage is random, regular or clumped. \(k\) varies between about 0.3 for erect foliage and 1.0 for canopies comprising horizontally disposed leaves. In general terms,
\[ F = 1 - e^{–k\mathrm{LAI}} \tag{12.5} \]
Unlike closed canopy forests or a continuous crop cover, sunlight interception by discontinuous canopies consists of two parts, a fraction determined by the overall form and extent of the canopy (\(F_\mathrm{max}\)) and a leaf area part that determines how close a particular orchard approaches the maximum interception attainable. \(F_\mathrm{max}\) would be reached if LAI was infinite. Accordingly, Equation 12.5 can be rewritten for discontinuous canopies as Equation 12.6, where \(L' = \mathrm{LAI}/F_\mathrm{max}\) (Jackson and Palmer 1979).
\[ F = F_\mathrm{max} (1 - e^{–kL'}) \tag{12.6} \]
This relationship for a discontinuous canopy contrasts with that for the continuous canopy (Equation 12.5) where an infinite LAI would cause complete exclusion of light. When light interception by two of the orchards in Table 12.1 is plotted as a function of LAI (Figure 12.29) and compared with a continuous canopy with the same extinction coefficient, light interception by either orchard approached an asymptote well short of infinite LAI.
Orchards were either a continuous canopy (\(F_\mathrm{max}\) = 100%), hedgerows 2 m tall, with 3 m row spacing, 1.5 m thick at the base and 0.5 m at the top (\(F_\mathrm{max}\) = 74%) or hedgerows 1 m tall, with 5 m row spacing, 1.5 m thick at the base and 0.5 m at the top (\(F_\mathrm{max}\) = 39%). Note that light interception depended on both LAI and \(F_\mathrm{max}\), so that the same light interception can be achieved with a very different LAI or the same LAI can be rearranged to intercept a different amount of sunlight.
Light interception by discontinuous orchard canopies can, therefore, be increased by increasing LAI, but a far more effective strategy is to increase canopy extent. Put another way, it is better to use orchard design to increase \(F_\mathrm{max}\) and thereby intercept sunlight that would otherwise fall into clear alleyways, rather than intercept sunlight that had a good chance of being absorbed by other foliage. Knowing the physical dimensions of the canopy, \(F_\mathrm{max}\) can be calculated from a mathematical model. \(F_\mathrm{max}\) complex shapes can be determined from CAD graphics.
As for annual crops and forest canopies, there is also a linear relationship between biomass production and canopy light interception for horticultural crops, even though the horticultural yield is measured as fruit weight rather than biomass (Figure 12.30. Thus in all managed cropping systems, a universal relationship between canopy light interception and yield or biomass production and the LAI, whether based on continuous or discontinuous canopies, underpins this relationship through determining the extent of light interception by the canopy.Although the upper limit of orchard dry matter production is set by light interception as outlined above, crop composition is determined by sunlight distribution within the canopy, and shade can reduce fruit quality. This is a general phenomenon among perennial fruit crops and has been reported for apple, citrus, peach, cherry, kiwifruit and raspberry. In apple, for example, fruit from more shaded regions are smaller, and have less red colour and lower soluble solids. There are also effects on apple quality after storage, with shaded fruit of Cox’s Orange Pippin tending to suffer more from shrivel and core flush and less from bitter pit than fruit from well-exposed regions of the tree. Fruit of satsuma mandarins grown in shaded regions of the trees are smaller, with a higher acid content and lower sugar content than fruit grown in well-exposed parts of the canopy.
With both peaches and cherries, shading has been found to reduce fruit colour, soluble solids and fruit size and delay abscission and maturity. Kiwifruit from shaded regions of the canopy, or individual fruit which has been artificially shaded during the season, show reduced fruit fresh weight, soluble solids, firmness and chlorophyll concentration in the mesocarp compared to well-exposed fruit. With grapevines, too, shaded fruit have generally lower sugar, total phenol and anthocyanin but higher pH, malate, potassium and titratable acidity than fruit grown in well-exposed positions in the canopy. These differences in grape quality have carried through to the wine quality made from the grapes. A consequence of decreased sugar levels in shaded grapes is a delay in harvesting. This may put crops at risk in climatically marginal regions.
Generalisations on sunlight and fruit quality have been derived from shading experiments on whole trees or parts of trees, or from correlation studies of fruit quality and irradiance in different parts of the canopy. Consequently both fruit and foliage have been subjected to reduced light, but some features in grapes and apples, such as anthocyanin development, vary according to illumination of the fruit itself. With grapes, cluster shading reduces fruit anthocyanin and total soluble phenolics, while leaf shading results in smaller berries with lower glucose and fructose content.
Shade within orchard trees can arise from self-shading or from adjacent trees or shelterbelts. Light levels in midsummer within well-spaced, traditionally pruned large peach trees can drop to 4–15% of incoming radiation. Sour cherry trees tend to be more dense, and light levels as low as 2–4% have been reported. Vigorous grape canopies can be very dense, with irradiance within the canopy reduced to less than 1% of ambient. With such dense canopies, between-row shading will be unacceptable if the ratio between canopy height and clear alleyway spacing exceeds 1:1 (Smart 1989). With the much more open canopies of apples, such ratios may be less useful, as acceptable light distribution within the trees can be obtained over a wide range of ratios of canopy height to clear alleyway spacing. With apple canopies, a ratio between actual light interception and \(F_\mathrm{max}\) may be more useful. If this ratio exceeds 0.7 then self-shading is probably excessive.
Overall effects of shade on fruit quality are very clear, but processes responsible are not so clear. Shade reduces PAR and, therefore, local photosynthetic activity; but canopy shade also reduces temperature (Greer and Weedon 2012) and changes wavelength distribution of transmitted light. Leaves absorb strongly in the visible part of the spectrum (400–700 nm), but have a high transmittance and reflectance to wavelengths in the near infrared (see Figure 12.1 at start of this chapter). This difference in light quality between incident and transmitted sunlight is usually expressed as a red/far-red ratio, that is, a ratio between irradiance around 660 nm compared with 730 nm wavebands. These wavebands correspond to peaks of absorption by phytochrome (Chapter 8), and are strongly implicated in developmental responses to canopy shade. Correlated changes in irradiance and the red/far-red ratio of canopy light make it difficult to separate the effects of changing irradiance from the effects of a change in the red/ far-red ratio, but it is likely that changes in berry composition are due to combined effects of irradiance and red/far-red ratio.
Due to the perennial nature of fruit trees, environmental conditions in one season can have carry-over effects on growth and development during the subsequent season. In seasonally deciduous trees and vines, flowers that emerge each spring were actually initiated during the previous growing season, and conditions prevailing at that time will have influenced the extent and intensity of flower bud differentiation. In temperate horticulture, flower initiation is not a continuous process but may be restricted to several weeks during mid to late summer, followed by several months of continuing development before passing into dormancy over winter. Floral development is then completed during the following spring ahead of flower emergence. In addition to changes in fruit quality of current crops, canopy shade during summer will also reduce flower bud initiation and differentiation.
Flower bud reduction due to shading seems to be a general phenomenon in perennial fruit crops and has been reported for apple, pear, apricot, peach, coffee, cacao and grape. With grape, the number and size of cluster primordia generally increases with increasing light. In this case, plants are responding to light quantity rather than quality (expressed as changes in the red/far-red ratio). Cultivars differ in their response to shade. Sultana (syn. Thompson Seedless) requires approximately 30% full sun for cluster initiation compared to 10% for Rhine Riesling. Timing of shading is also important for grapes. Shading in the period during bud development of grape in late spring has the greatest negative effect on flower initiation.
In citrus, shading may not of itself reduce flowering but can induce heavy fruit abscission. Similarly, shading whole apple trees 14–28 d after full bloom can result in very heavy fruit loss. Two days shading with shade cloth transmitting 8% light was sufficient to reduce fruit set by 80% (Byers et al. 1991). So effective is this treatment that deep shade could work as a non-chemical fruit thinner! In sour cherry, fruit set is adversely affected by light levels within the canopy below 20%, and flower bud initiation requires an irradiance of 15–20% full sun. Shading of whole vines or shoots of kiwifruit during summer can reduce flowering the following spring. Again, as with grapes, changes to the red/far-red ratio did not alter the flowering response of kiwifruit vines to total irradiance. Yields of kiwifruit vines next to shelterbelts are significantly lower than those from vines in the centre of fruit blocks, due presumably to a reduction in flowering as a result of shade from the shelterbelt. This can depress yield by two thirds.
While shading generally reduces fruit quality, excess solar radiation can lead to serious downgrading of fruit quality. Apples, grapes and kiwifruit suffer from sunburn, particularly where the fruit has been growing in the shade and then is thrust into full sunlight by movement of branches due to weight of fruit or to summer pruning or defoliation removing foliage that previously shaded the fruit. This tends to be a much larger problem in regions of high solar radiation receipt than cloudy areas.
Perennial fruit orchards do offer great potential for modification of light and temperature environments within canopies by pruning, tree training, tree size, row spacing and row orientation. Because of the great variety of ways of arranging the foliage in space, a number of models have been written to examine the relative importance of some of these factors in influencing not only light interception (Table 12.1) but also sunlight distribution within the canopy (Palmer 1989; Wagenmakers 1991), as this can have such a profound effect upon the quality of the fruit as already outlined.
In addition, field experiments to examine these effects are very expensive and time consuming to set up and run, so models offer a very economical way of examining light interception and distribution within innumerable canopy forms. As there is often a large dollar premium for quality, the economic success of any production system is largely determined by the yield of high-quality fruit, rather than the total yield. That does not mean, however, that high total yield and high-quality yield are mutually exclusive. With grapevines, Smart (1989) has consistently argued that high yields of high-quality grapes are achievable via correct canopy management.
Highly productive orchards and vineyards, therefore, have a balance of vegetative and reproductive growth, with fruiting zones maintained in a high light environment. Examples of how this has been achieved will now be presented for apples, peaches and grapes. In each case only one system is described, although there are many other successfully managed systems, particularly in vineyards.
In New Zealand, Tustin et al. (1990) have sought to combine requirements for precocity, high yields of high-quality fruit and good light penetration into the canopy in their slender pyramid tree form. This has been developed with trees on semi-vigorous rootstocks at a tree density of about 700 trees per hectare. The form is basically a central leader tree, that is, one central vertical trunk, on which are borne the fruiting branches. Early tree management is aimed at developing a permanent, strong, spreading basal tier of four to five branches emanating from the central leader at wide angles for strength and to encourage early fruiting. Pruning is minimal in the early years, with unwanted shoots being removed during the growing season when they are still small. The upper part of the tree is developed as an open, well-spaced arrangement of whorls of shorter branches arising directly from the central leader. These upper branches are removed when they become too large or pendulant and are replaced by natural regrowth. Each tree is maintained in an overall pyramidal form to encourage light penetration into all parts of the canopy. Yields of Royal Gala apples, for example, have been over 50 t ha–1 in the third year rising to over 100 t ha–1 by year six.
Trees on a Tatura trellis (Figure 12.27) are trained into two planar canopies inclined at an angle of 60º from the horizontal, and held by a trellis system of posts and wires. The system is designed to encourage precocity by using 2,000 trees per hectare and rapidly filling the trellis structure with fruiting branches. Within each arm, the canopy itself is shallow to encourage good light penetration. Summer pruning of unwanted, upright vegetative growth is practised to maintain a high, uniform light interception environment along each arm. Vegetative growth has also been successfully controlled in the dry environment of Tatura by regulated deficit irrigation.
The Tatura trellis is also designed for mechanical picking. A rigid and shallow canopy ensures that fruit can fall onto the catching frame with minimal chance of damage. As peaches bear fruit on one-year-old wood, yields of Golden Queen on Tatura trellis in year two have reached 28 t ha–1 rising to a maximum of 86 t ha–1 by year four (van den Ende et al. 1987). Other stonefruit and pomefruit (pipfruit) have also been grown successfully with this management system.
Traditional pruning of grapevines was aimed at producing a limited number of moderate-sized clusters of berries to facilitate hand picking. Consequently, pruning removed large amounts of young wood so that growth of vigorous shoots was stimulated. With the advent of mechanical picking for wine grapes, however, this constraint has been removed as the harvesters are capable of efficiently removing the berries from large and small grape bunches. This has consequently brought about new grapevine pruning and training techniques, particularly in regions with long growing seasons, irrigation and high vigour such as Australia, Chile and California.
Minimal pruning of cordon-trained vines (MPCT) is one such example from Australia. Each vine is initially developed conventionally with two or four permanent horizontal arms or cordons. From year three, vines are minimally pruned — low hanging canes are removed. Consequently MPCT vines carry far more buds over from one year to the next, but competition between these growing points results in shorter shoots, with shorter internodes, and higher yields made up of a large number of small clusters of berries well exposed to the light. The resulting wine quality is high and comparable with that from conventionally pruned vines.