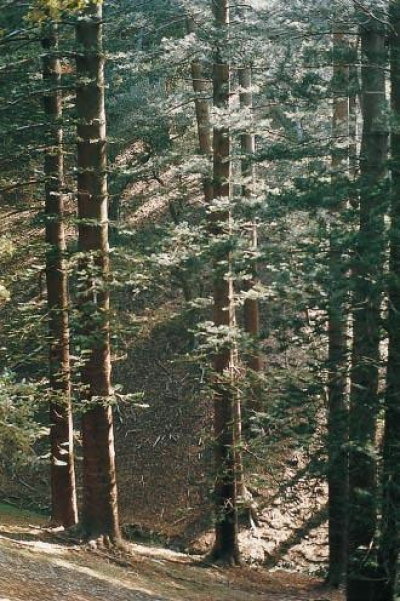
Shafts of sunlight penetrate a natural forest of Norfolk Island pine (Araucaria heterophylla). (Photograph courtesy D.H. Ashton)
Chapter editor: Dennis H. Greer
School of Agricultural and Wine Sciences, Charles Sturt University, Australia
This Chapter is updated from the original chapter (1st edition) from Sharon Robinson, Jenny Watling, Dennis Bittisnich, Shu Fukai, Chris Beadle, Mike Clearwater and Paul Kriedemann.
Plants have adapted to an extraordinarily wide range of light environments, from the deep shade of rainforest understoreys and underwater habitats to the high-radiation environments of deserts and mountain tops. Exploitation of a wide diversity of habitats is possible because plants have evolved various mechanisms to optimise their use of sunlight. Many plants also exhibit great plasticity in their response to changes in light availability within a particular habitat. This potential for acclimation enables plants to exploit more variable environments than plants with a narrower range of responses to light.Terrestrial ecosystems are both sustained and regulated by sunlight: sustained in massive ways by photosynthetically active radiation, but regulated in subtle ways by other wavelengths. Wavelengths most effective for photosynthesis occupy a band between about 380 and 720 nm. A wider band from about 350 to 800 nm spans the action spectra for other crucial responses in plant growth and reproductive development that are also light regulated. These include seed germination, tropisms, morphogenesis, pigmentation, and photoperiodic responses such as floral initiation (topics covered in Chapter 8 - environmental effects on plant development).
The outcomes of these two light-dependent categories differ by many orders of magnitude in terms of energy flow within a plant community. In one case, a flow of radiant energy is converted into chemical energy and stored as biomass; in the other, miniscule levels of radiant energy trigger shifts in gene expression and consequent developmental responses. Nevertheless, each category is mediated by pigment systems that transduce solar energy into highly ordered chemical forms: biosynthetic systems in photosynthesis, triggering systems in photo-morphogenesis (light-mediated development, where plant growth patterns respond to the light spectrum).
The earth’s atmosphere attenuates solar radiation in highly selective ways (Figure 12.1). Substantial amounts of infrared energy (between about 850 and 1300 nm) are absorbed by CO2, ozone and especially by water vapour, while ozone is principally responsible for a cut-off in ultraviolet radiation below about 300 nm. Attenuation of discrete bands of infrared radiation by water vapour is especially noticeable (right side of figure), while absorption of ultraviolet by stratospheric ozone is also of special significance for terrestrial organisms. Cloud light is especially rich in visible wavelengths with a peak around 500 µm. Our atmosphere thus represents a ‘window’ through which visible wavelengths pour onto the earth’s surface, and terrestrial life forms have evolved with attributes that are a direct consequence of this spectral composition.
Selective filtering of wavelengths either side of the visible spectrum is crucial. Ultraviolet radiation is comprehensively absorbed by biological ingredients, especially proteins, RNA and DNA and pigments. Because energy per quantum increases with the decrease in wavelength, ultraviolet radiation imposes a heavy load of energy on biological components with attendant disruption. Similarly, wavelengths beyond the visible spectrum, though less energetic, are still damaging because tissue water absorbs infrared radiation. All life processes operate within an aqueous milieu, so that the absorption properties of water molecules would put cellular function at risk if sunlight was not also attenuated with respect to infrared radiation by the atmosphere.
Between these two extremes stands the visible spectrum, and it is no coincidence that all manner of biological systems have evolved to make effective use of this narrow band of solar radiation. Vegetation shows strong attenuation of visible wavelengths that drive photosynthesis, but transmit near-infrared radiation that would otherwise heat leaves. Vascular plants are a case in point, where canopy, leaf and chloroplasts have all adapted to the light climate with features that optimise their use of sunlight. These include mechanisms to deal with excess radiation.
Photosynthetic efficiency in low light confers a selective advantage on shade-adapted plants, but also renders them especially vulnerable to full sun. Accordingly, such species have evolved remarkable features for photoprotection. Their acclimation to sun and shade, together with properties of sun-loving plants, thus reveal an extraordinary plasticity in the photosynthetic apparatus of vascular plants (Section 12.1). Even increased UV-B radiation, commonly associated with global change, and the so called ‘ozone hole’ over Antarctica, elicits responses that offer photoprotection to plants (Section 12.6).
Aside from the biological hazards, solar radiation obviously sustains global photosynthesis, and close quantitative relationships exist between energy absorption and biomass production. Such relationships are especially well defined for managed communities, with cropping, plantation forests and horticulture providing clear examples (Sections 12.2, 12.3, 12.4). Canopy architecture is a major determinant of sunlight interception, and hence production of biomass. Canopy pruning in horticulture are examples of enhanced productivity through increased interception of light. In such cases, light-dependent regulation of plant development assumes prominence because tree and vine canopies are shaped for both overall interception of light as well as maximum fruitfulness.
A note on units: Visible wavelengths of sunlight can be represented as either a quantum flux or a radiant energy flux. Quantum flux is regarded here as synonymous with 'photon irradiance' (Q) and has units of µmol quanta m-2 s-1 ('µmol quanta' rather than 'µmol photons' because the quantum energy derived from photons drives photosynthesis). For the sake of making a clear distinction from quantum flux, radiant energy flux is simplified to 'irradiance', and for present purposes, irradiance coincides with photosynthetically active radiation (PAR). Irradiance is then expressed as joules (J) per square meter per unit time. Depending on the application, time can span seconds, days or years, and is then coupled with either joules, megajoules (MJ) or gigajoules (GJ).
In low light, plants need to absorb maximum light for photosynthesis if they are to survive. In high light the problem is reversed. Plants need to maximise their capacity for utilising their abundant light energy. At the same time, the plants have to deal with excess sunlight when their photosynthetic capacity is exceeded. As a consequence of such unrelenting selection pressures, plants have evolved a variety of features that optimise light interception, absorption and processing, according to the light environment in which they had evolved and adapted (Figure 12.2). Adaptation implies a genetically determined capability to adjust attributes, i.e., acclimate to either sun or shade. Such acclimation calls for adjustment in one or more attributes concerned with interception and utilisation of sunlight. Common features of either sun or shade plants are outlined below, and the advantage to plants growing in different light environments is discussed. Field applications are illustrated with examples of sun/shade acclimation and sunfleck utilisation in rainforest plants.
Initial steps of photosynthesis involve interception and absorption of photons by photosynthetic organs; subsequent steps are involved with utilisation or dissipation of the absorbed quantum energy. Interception of light varies according to size, angle, orientation and surface features of the photosynthetic organ(s) and is also influenced by the arrangement of photosynthetic tissue within those organs (Figure 12.2).
Many plants can change their leaf angles and orientation in response to a change in light. For some, to increase interception while for others, to avoid high light. A good example of optimising light interception through leaf movement is given by Oxalis oregana, an understorey herb of redwood forests in western USA (Figure 12.4). This plant is able to track sunlight on dull days, but can change leaf angle from horizontal to vertical in only 6 min, if exposed to full sunlight. In this way, leaves can maintain maximum photosynthetic rates under a variety of light conditions but can avoid photoinhibition of photosynthesis by leaf folding. Omalanthus novo-guinensis, an Australian rainforest plant, can also change leaf angles within about 20 min in response to full sunlight (Watling et al. 1997b).
Another way of reducing light capture is a change in leaf-surface properties. Many plants in high light environments have a high reflectance of their leaves from a coat of hairs or wax or even salt crystals. Cotyledon orbiculata, a crassulacean acid metabolism (CAM) plant from southern Africa, produces a wax coating on the leaves. Plants grown at high light produce copious quantities of white wax which reflects 60% of incident light whereas plants grown in low light produce very little wax and leaf reflectance drops to 9% (Figure 12.5). Young eucalypt leaves also produce wax, while leaves of Celmisia longifolia, the snow daisy of the Australian Alps, are covered in a thick layer of silvery fibres. In these instances, plants are avoiding high light by creating their own shade, but does leaf anatomy adjust to environments where light is limiting?
Epidermal cells in some rainforest shade-adapted species are shaped to enhance light capture by acting as a lens. The optical properties of such cells focus incident sunlight into the layer of photosynthetic tissue just below the epidermis, reducing light lost due to reflectance and transmittance.
Light interception can also be regulated at a tissue and organelle level. Photosynthetic tissue can be concentrated equally on both sides of a leaf (isobilateral) to maximise light absorption from either side, or preferentially on one side (dorsiventral) as is common in species where leaves are predominantly horizontal.
Chloroplast density and location within leaves is also sensitive to the light climate, and energy capture varies accordingly. Alignment along vertical cell walls will reduce overall absorption of incident light, and in Oxalis leaves absorbance can be reduced 20% when chloroplasts align less to the horizontal and more to the vertical walls of mesophyll cells.
Once sunlight has been intercepted by an assimilatory organ, photon absorption then depends on the extent and nature of light-absorbing pigments in the photosynthetic tissues. In terrestrial plants, the major light-absorbing pigments are chlorophylls a and b plus a range of carotenoids which can act as accessory pigments. Compared with high-light plants, plants grown in low light tend to allocate relatively more resources to their light-harvesting pigments and the associated proteins than to the enzyme Rubisco and other soluble proteins involved in CO2 fixation. This shift in allocation of nitrogen-based resources can be accompanied by marked changes in leaf anatomy, especially depth of mesophyll tissue (see Case study 12.1) and reflects a need for increased efficiency of light absorption when sunlight is limited.
There are also differences in chloroplast structure between plants grown in low light and high light. Shade chloroplasts tend to be larger than those found in sun plants. They also contain more thylakoid membranes which show higher levels of randomly arranged granal stacking into appressed regions, as shown by the extreme development of grana in Figure 12.6. The higher proportion of appressed to non-appressed membranes found in shade chloroplasts is the result of increased photosynthetic system II (PSII) and antenna (LHCII) content. LHCII is thought to be involved in thylakoid appression and formation of granal stacks. Plants grown in low light also tend to have lower Chl a/b ratios. Chlorophylls a and b are both associated with the light-harvesting antennae, while only Chl a is found in the reaction centres. A lower a/b ratio, therefore, reflects an increase in LHCII complexes relative to reaction centres (see Chapter 1, Section 1.2).
In addition to differences in leaf anatomy and chloroplast fine structure, energy derived from absorbed sunlight is processed in ways that differ subtly between shade-grown and sun-grown plants. In high light, there is a requirement for greater capacity in both the light and CO2 fixation reactions of photosynthesis. Photosynthesis–light response curves for shade and sun plants (Figure 12.7) illustrate such differences. The initial slope of each light response curve represents the quantum or photon efficiency of photosynthesis. This is the same for sun and shade plants. The reason it does not change is that the efficiency of the light reactions is the same irrespective of how much light has been received during growth (i.e. eight photons are required for the evolution of one molecule of O2 and fixation of one molecule of CO2 in all plants).
Figure 12.7 Photosynthesis-light response curve for typical shade and sun plants, showing relationships between photosynthetic rate and absorbed light (expressed as a photon irradiance). Dashed lines are extrapolations of initial linear slopes where photosynthesis is light limited, and represent quantum yield (moles of O2 evolved per mole quanta absorbed). (Original data courtesy S.A. Robinson)
However, sun plants tend to have a greater capacity for photosynthetic electron transport (greater abundance of transport components such as Cyt b559, Cyt b563, Cyt f and plastoquinone). They also have a greater capacity for ATP synthesis per unit of chlorophyll compared with shade plants. Taken together, these capacities of sun plants allow more sunlight to be processed into ATP and NADPH for use in CO2 assimilation and other synthetic events. Such capacity is also matched by a greater investment in enzymes of the photosynthetic carbon reduction (PCR) cycle, resulting in a higher light-saturation point and a higher maximum rate of photosynthesis (Pmax) for sun plants (Figure 12.7). As a further distinction, sun leaves tend to be thicker and have more cell layers. They also have higher stomatal conductances to facilitate rapid uptake of CO2.
A higher photosynthetic capacity in sun plants does, however, incur some costs. The sun leaves tend to have higher respiration rates which increases the light-compensation point relative to shade plants (Figure 12.7). Higher respiration rates probably result from (1) increased carbohydrate processing in high light, (2) increased costs of constructing sun leaves and (3) a higher cost of maintaining sun leaves. Further details on maintenance costs are given in Chapter 5, Section 6.5.
Greater transpiration is a further cost of the higher photosynthetic capacity as a result of higher stomatal conductance. Sun plants often respond to the greater transpiration by increasing their root : shoot ratios. Under conditions where water is limiting, however, stomatal conductance may be reduced, sacrificing photosynthesis in favour of slower transpiration.
In the light-response curves for photosynthesis (Figure 12.7 above), photosynthesis is regarded as light limited in the initial linear region of the curve. However, at higher photon irradiances once the light-saturation point (that is, the maximum rate of photosynthesis) has been reached, further increases in light will exceed the energy-utilising capacity of that photosynthesising leaf. Refer to the dashed lines in Figure 12.7 which represent a continuation of the initial rate of photosynthesis (quantum yield of photosynthesis) and demonstrates the actual light absorption. The extent to which this absorbed light is not ‘gainfully employed’ for photosynthesis is set by Pmax (the light-saturated rate of photosynthesis in normal air). At low light (< 100 µmol quanta m–2 s–1), both sun and shade leaves use more than 80% of the absorbed light for photosynthesis. However, once Pmax has been reached, all additional absorbed light is in excess of that which can be used in photosynthesis.
Since shade plants have a lower Pmax than sun plants, they experience more excess light at a given photon irradiance above saturation. Additional stresses such as drought, nutrient limitation or temperature extremes can also lead to a reduction in Pmax and thus increase the probability of plants being exposed to excess light. However, even the most hardy sun plant will reach Pmax at less than full sunlight (incident beam normal to leaf surface). At that level (say, 1000 µmol quanta m–2 s–1), approximately 25% of absorbed energy is used to drive photosynthesis, but at full sunlight (c. 2000 µmol quanta m–2 s–1) as little as 10% is used (Long et al. 1994). Individual leaves on plants growing in full sun commonly experience such excess light intensities. This is potentially damaging, and plants adapted to full sunlight have evolved a number of mechanisms for either avoiding excess light or for dissipating the excess absorbed energy.
Figure 12.8 As photon irradiance is increased, utilisation of energy gives way progressively to dissipation of energy. Photosynthetic events shift from photochemistry, to external and then internal photoprotection and finally to photodamage. Their comparative importance for shade leaves and sun leaves is indicated. Photoprotection is especially well expressed in sun leaves acclimated to additional environmental and biotic stresses. (B. Demmig-Adams and W.W. Adams, Annu Rev Plant Biol 43: 599-626, 1992)
Shade plants have an even greater need to dissipate excess light interception because they absorb more light (more chlorophyll per unit leaf mass), but need less light to saturate photosynthesis. Prolonged exposure of plants to excess light induces photoprotective processes that reduces the photon yield of photosynthesis, but Pmax remains unchanged (curve A). However, further exposures to excess light will result in both photon yield and Pmax being reduced (curve B). The photosynthetic rate is then reduced at all light levels as a consequence of the photoprotection.
Photoprotection is normally sufficient to cope with light absorbed by leaves; the more extreme photodamage only occurs when the capacity for photoprotection is exhausted. Photodamage is manifested as a decline in both photon yield and Pmax, and recovers very slowly (hours to days), whereas photoinhibition and photoprotection recovers much faster (minutes to hours). Severe photodamage results in bleaching of pigments and damage to membranes (photo-oxidation) and may lead to tissue death.
Consider the alternative fates of light energy absorbed by a leaf and their relevance to photoprotection, photoinhibition and photodamage (Figure 12.10). Although blue light has higher energy and causes excitation to a higher excited state, this energy is quickly lost as heat, and chlorophyll molecules drop to a lower excited state. Utilisation of energy from excited chlorophyll molecules results in either assimilatory or non-assimilatory photochemistry, thermal dissipation or release of light of a longer wavelength (fluorescence). The proportion of absorbed energy consumed by these different processes dictates their comparative significance, and in order of importance as protective devices they are:
Most of the NADPH and ATP formed during photosynthetic energy transduction is stored as stable photosynthetic products. Some is consumed in photorespiration and nitrate reduction. Because these non-assimilatory processes also utilise NADPH and ATP, they help reduce the need for photoprotection. The Mehler reaction, in which electrons flow to O2 via photosynthetic system I (PSI) (Chapter 1, Figure 1.10), still supports electron flow and thus might also reduce a need for photoprotection.
However, if photochemical capacity is exceeded by incoming energy, a plant will engage photoprotective mechanisms which increase the amount of energy dissipated as heat. This non-photochemical conversion of light energy is thought to occur in the PSII antennae and involves a group of pigments known as xanthophylls and includes violaxanthin, antheraxanthin and zeaxanthin (Figure 12.11). These are a special group of carotenoid pigments which undergo interconversion in response to excess light. Energy is dissipated in the process. In low light, violaxanthin predominates, but when light is in excess, conversion to zeaxanthin via antheraxanthin occurs. This conversion requires a low pH, ascorbate and NADPH. Such conditions exist in the lumen of chloroplasts in high light. Zeaxanthin, and possibly antheraxanthin, provide photoprotective thermal dissipation of the excess light energy. When light levels are no longer excessive, zeaxanthin slowly converts back to violaxanthin via antheraxanthin (Figure 12.11). Total pool sizes of the xanthophyll pigments increase with increasing exposure to excess light. Sun plants can have three- to four-fold larger pools of violaxanthin, antheraxanthin and zeaxanthin than shade plants and the presence of other stresses can also result in increases in pool size.
Internal differences between sun and shade leaves with respect to energy dissipation are also apparent in the different patterns of attenuation of light through mesophyll tissues, such as in succulent CAM plants such as Cotyledon orbiculata. There, xanthophylls are mostly found in outermost cell layers where the light environment is strongest. If the reflective wax coating is intact, no internal photoprotection is required at the growth irradiance and there is no zeaxanthin formed. However, if the surface wax (external photo-protection) is removed by hand, internal photoprotection is then needed and zeaxanthin appears in the outermost layer (Figure 12.12).
Overall activity of the xanthophyll cycle varies with mesophyll irradiance, while the concentration of particular components also varies according to tissue depth. Changes in concentration of the various components of the xanthophyll cycle are shown here for successive (1 mm) layers in thick leaves of C. orbiculata, with and without their wax coating. In waxed leaves at the end of a dark period (top figure, Figure 12.12) xanthophyll cycle pigments are represented mainly by violaxanthin (V) with a small amount of antheraxanthin (A). After 6 h exposure to high light (middle figure, Figure 12.12) a small amount of violaxanthin is converted to antheraxanthin, but no zeaxanthin (Z) is formed, indicating that the natural wax coating on these leaves is protecting chloroplasts from excess light. Physical removal of surface wax (brushed leaves in bottom figure, Figure 12.12) results in zeaxanthin production within the uppermost layer of leaf tissue but not in deeper tissues or lower surfaces. Restriction of this xanthophyll cycle component to the top section confirms that zeaxanthin accumulation is a response to excess light.A seed germinating in a rainforest understorey starts life in a low light environment. This will not present major problems to an obligate shade species which cannot tolerate strong sunlight; such species have adapted to life in an understorey. However, many rainforest species are better described as either shade tolerant (i.e. able to germinate and persist in low light, but requiring higher light to reach maturity) or shade intolerant (unable to germinate or grow in low light). In successional terms, shade tolerance is a feature associated with climax species and shade intolerance with pioneer species.
Shade-tolerant species can persist as seedlings in the understorey, often for years, while still being able to respond to an increase in light availability when it occurs.
By comparison, shade-intolerant (early-successional) species can only germinate and grow where there is ample sunlight, and consequently they tend to occur in wide gaps and on forest edges. Wide gaps are relatively rare in old-growth rainforests which have been undisturbed by logging or slash and burn agriculture. Shade-intolerant species are unable to maintain a positive carbon balance when growing in low light. The change from a high light to a low light environment requires a change in allocation of plant resources as described in the quantitative growth chapter (Section 6.2). Shade-intolerant plants are unable to make this change and are burdened with the higher costs of construction and maintenance of leaves better suited to strong sunlight
Shade-intolerant species tend to produce numerous small seeds throughout the year which are widely dispersed. Their seeds are also able to remain viable for long periods (years) by going through a period of dormancy. This is often broken by high temperature or strong direct sunlight with a high ratio of red to far-red irradiance (R:FR ratio decreases with sunlight attenuation through canopies). Such environmental cues for germination are all experienced in wide gaps. Following germination, seedlings show rapid growth to maturity, allowing them to become well established in a gap before other slower growing species. These characteristics increase the probability of success for shade-intolerant species in the heterogeneous light environment of a rainforest.
Shade-tolerant species, on the other hand, have evolved a different suite of characteristics. They tend to produce a few large seeds which are generally not well dispersed, with little or no dormancy. However, the seeds have the ability to germinate in low light and persist in the understorey as seedlings for years. A rarity of gaps and a lack of dormancy found in most shade-tolerant species increases the probability of establishing in a low-light understorey environment. In situations like this, the larger seed provides seedlings with a reserve which they can draw upon during early establishment. In rainforests, tree seedlings survival in understorey habitats is positively correlated with seed size, especially in the first few months following germination.
Following establishment in the understorey, seedlings of shade-tolerant species may have to wait a long time before a gap appears overhead. Many species succumb to attack from herbivores or pathogens or may be crushed by large animals (including humans!). Those that do survive must be able to acclimate to the new conditions arising on gap formation; their ability to do this will depend on the nature of the new microclimate and the acclimation potential of each species.
Emergent trees of tropical rainforests have to endure strong sunlight, and leaves comprising the crowns of such trees will have acclimated to full sun. In young-growth forests, canopy emergents are early-successional fast-growing species that are adapted for fast growth in full sun on large disturbances. Such species represent an initial phase in forest dynamics that might last 10–20 years. By contrast, in old-growth forests, early-successional species have long since completed their life cycles, and will have been replaced by later-successional species whose seedlings initially tolerated deep shade on the forest floor, but now endure full sun as canopy emergents. Such remarkable plasticity is an adaptive feature of late-successional species and involves sun/shade acclimation by individual leaves.
The differences in growth rate of early-successional fast-growing species versus later-successional and shade-adapted species is illustrated in Figure 12.13 by two rainforest species that are important in the timber industry: the sun-loving red cedar (Toona australis) and the shade-adapted tulip oak (Argyrodendron sp.).
A detailed study of three rainforest species from North Queensland was conducted by Thompson et al. (1992a, b). The red cedar Toona australis (an early-successional species) and two species of tulip oak Argyrodendron (late-successional species) showed different acclimation potentials when grown under a range of light conditions (Figure 12.14).
When grown under high light, 535 µmol quanta m-2 s-1,which is typical of average canopy radiation in a tropical rainforest, T. australis achieved a higher Pmax and light-saturation point than either of the Argyrodendron species. However, T. australis was more sensitive to nutrient levels, being unable to acclimate to the same degree in low-nutrient compared to high-nutrient regimes. Moreover, fast growth in T. australis was greatly promoted by a positive light x nutrient interaction on leaf expansion and photosynthetic capacity; adaptive features with a clear selective advantage on open sites where soil disturbance liberates nutrients.
Figure 12.14 Photosynthesis versus light response curves for seedlings of a shade-adapted rainforest tree species (Argyrodendron) and a sun-loving tree (Toona australis). Seedlings were grown under factorial combinations of weak, medium or strong light (shown left to right) × either high or low nutrient supply (solid lines with filled symbols, and dashed lines with open symbols respectively). (W.A. Thompson et al., 1992b)
Toona (sun loving) produced a much greater depth of mesophyll tissue under strong light compared to weak light (Figure 12.15b), which accounted for enhanced photosynthetic capacity. Argyrodendron sp. (shade adapted) was much less responsive to photo irradiance during growth, producing consistently thicker leaves regardless of light level, but with lower photosynthetic capacity.
These adaptations result in the more vigorous growth of Toona than Argyrodendron at high light.
Formation of gaps provides an important opportunity for many rainforest plants to escape from the dim understorey environment and reach maturity as canopy trees. Rainforest habitats actually present a continuum of light availability ranging from almost total shade (diffuse light) through intermediate levels of direct and diffuse sunlight to a wide gap where direct sunlight is received for most of a day. Input of direct sunlight beneath a closed canopy can be surprisingly high because of sun patches and more transient sunflecks. Sun patches occur when small and variable openings in the overlying canopy permit direct sunlight to penetrate to the forest floor, resulting in the familiar patchwork of sunlight and shade which can be seen in any understorey on a clear day (Figure 12.16).
Competition for light is intense, and a sunfleck shown passing over this tiny population would be providing a much-needed source of sunlight, namely a few minutes of around 1,500 µmol quanta m-2 s-1 compared with background photon irradiance of around 150 µmol quanta m-2 s-1.
Patches of sunlight move across the forest floor on bright days, and will illuminate any leaves or parts of leaves which lie in their path (Figure 12.17). Daily total photon irradiance on the occasion of the measurement shown in Figure 12.17 was 6.0 mol quanta m-2 d-1). The abrupt increase from a background level of around 50 µmol quanta m-2 s-1 to 1,750 µmol quanta m-2 s-1 energises photosynthesis, but is counteracted by increased leaf temperature, especially during prolonged exposures. Transpiration cooling is a significant component of heat budgets for such large leaves, so that adequate soil moisture is a prerequisite for continuing leaf gas exchange during a sunfleck.
Sunfleck frequency will be an additional factor for carbon gain during exposure to strong photon irradiance. Photosynthetic induction state diminishes to a minimum after 30 min in low light (see Figure 12.18 below) and some minutes are required to regain full capacity in strong light. Infrequent sunflecks are thus used with reduced efficiency.
Sunflecks and sun patches are of potential use to understorey plants for photosynthesis, but is this potential realised? Growth of understorey tree seedlings has been shown to be correlated with the amount of direct light received in sunflecks, and up to 60% of carbon gain in such environments has been attributed to this source. However, when compared with expected values based on the known steady-state response of plants to light, sunfleck utilisation is often below predicted values (Pfitsch and Pearcy 1989). Moreover, species vary in their capacity to utilise sunflecks. Watling et al. (1997a) measured the growth of four Australian rainforest species under simulated sunfleck regimes and showed that sunflecks contributed to growth in two species (Diploglottis diphyllostegia and Micromelum minutum), whereas the other two species (Alocasia macrorrhiza and Omalanthus novo-guinensis) were unable to make effective use of sunflecks.
Two components of a plant’s photosynthetic physiology will determine how the light in a sunfleck is used. Firstly, photosynthetic capacity (Pmax) will set a ceiling on the amount of light a plant can use. Secondly, a few minutes of illumination at least are needed for PCR cycle intermediates to reach critical levels, and this ‘induction requirement’ of photosynthesis determines how quickly a leaf can respond to an increase in photon irradiance.
Measured photosynthetic capacities of understorey plants are often low. When a leaf experiences a sunfleck, carbon fixation will increase to the point of light saturation. If an understorey plant could increase Pmax, it could utilise more light. But there are trade-offs. For example, higher respiration rates would increase the light-compensation point, and increase carbon losses during the low light periods separating sunflecks.
Regardless of photosynthetic capacity, the ability of a plant to use sunflecks is also affected by the induction requirement of photosynthesis. When a leaf that has been in low light for some time is exposed to an increase in photon irradiance, the rate of photosynthesis does not increase instantaneously to the new level. Instead, there is a gradual increase in assimilation which can take from 10–60 min for completion. This ‘induction period’ varies according to species as well as the induction state of the leaf concerned. Three different processes are involved; namely (1) buildup of PCR cycle intermediates and in particular ribulose-1,5-bisphosphate (RuBP), (2) light-dependent activation of Rubisco, and (3) light-dependent opening of stomata. Each of these processes follows a different time-course. Buildup of a metabolite pool is fastest (1–2 min), followed by Rubisco activation (2–5min) and finally stomatal opening (up to 60 min). Relaxation in low light is more protracted but generally occurs in the same sequence, leading to a decline in the induction state.
Thus, the longer a leaf has been in low light, the lower it’s the induction state. Figure 12.18 illustrates this for a leaf of Alocasia macrorrhiza, a plant common in rainforest understoreys and forest edges in eastern Australia. Fully-induced leaves were transferred to low light (10 µmol quanta m-2 s-1) for different lengths of time (up to 60 min). Their induction state was determined as the proportion of light-saturated photosynthetic capacity (Pmax) achieved within 2 min after return to saturating light. Induction loss in Alocasia, therefore, has a half time of about 25 min, but other species have been found to be either faster (e.g. Adenocaulon bicolor, an understorey herb from the redwood forests of western USA; Pfitsch and Pearcy 1989) or slower (e.g. Castanospora alphandii, a shade-tolerant tree from Australian rainforests; Watling et al. 1997a, b).
Chazdon and Pearcy (1986) showed that continuous light is not needed for induction to proceed. If leaves were subjected to a series of 60 s lightflecks (artificial sunflecks) separated by 2 min of low light, then induction state increased with each successive lightfleck. In nature, sunflecks are often clustered with a sequence of irregular bursts, separated by dull periods of variable duration. Under these circumstances, the state of induction might improve such that a plant will respond more rapidly to closing sunflecks in each sequence. This is supported by the data of Chazdon and Pearcy (1986), where the efficiency with which a sequence of lightflecks was utilised increased with successive lightflecks (efficiency was calculated as the actual amount of carbon fixed during a lightfleck relative to the amount predicted if there had been no induction period).
Carbon gain by fully induced leaves during lightflecks can exceed that expected, resulting in improved efficiency of light utilisation. This ‘intermittency phenomenon’ was noted by Kriedemann et al. (1973) in grapevine leaves exposed to high-frequency lightflecks. Similar investigations in a number of other species have also shown that such enhancement occurs only with short-duration lightflecks and is more prominent in fully induced leaves. Alocasia macrorrhiza showed improved efficiency of lightfleck utilisation by fully induced leaves only when lightflecks were of 40 s duration or less. Un-induced leaves needed lightflecks 10s or shorter (Chazdon and Pearcy 1986).
This apparent improvement in light use efficiency results from continued carbon fixation in low light (or darkness) following a lightfleck. During a short lightfleck, pools of triose phosphate and RuBP build up because carbon fixation runs transiently slower than the light reactions. This pool of PCR intermediates is then used for post-illumination CO2 fixation. In rainforest understoreys, where sunflecks are generally longer than a few seconds, this kind of enhancement is unlikely to be important. However, it may contribute significantly to carbon gain under crop canopies, where sunflecks are much shorter and more frequent.
One consequence of generally low photosynthetic capacities in understorey plants is a limited ability to process the light energy they absorb during strong sunflecks. This limited ability can also be exacerbated by a low induction state. Under these conditions, understorey plants will need to dissipate excess energy if they are to avoid photodamage. Field measurements of chlorophyll fluorescence from A. macrorrhiza show a decline in the quantum yield of photosynthesis (measured as Fv/Fm) during saturating sunflecks, indicating that photoprotective mechanisms are probably being engaged. Simultaneous assessment of the xanthophyll pigments shows that interconversion of violaxanthin to zeaxanthin is also occurring. After the sunfleck has passed, conversion of zeaxanthin to violaxanthin is extremely slow in species such as A. macrorrhiza, perhaps allowing a more rapid photoprotective response for subsequent sunflecks. However, quantum yield increases more rapidly than xanthophyll reconversion on return to low light, demonstrating a requirement for both high ΔpH and zeaxanthin for internal photoprotection to occur (Watling et al. 1997b).
Engagement of photoprotective mechanisms by shade-tolerant plants in an understorey environment may seem surprising, but serves to illustrate the extent of spatial heterogeneity in resource availability which is a feature of most habitats.
Barry Osmond
Plants often harvest more light than they can use in photosynthesis. When they are exposed to excess light there is an ever-present possibility of photoinhibition. This may happen when tree fall produces a rainforest gap and suddenly exposes seedlings adapted to life on a forest floor to sustained 10- or 20-fold increases in photon irradiance, or when water stress or low temperature restricts access to CO2 in sun plants. The photochemical efficiency of light utilisation (\(\phi_{PSII}\)) declines rapidly, and in most cases reversibly. Usually the excess light is wasted as heat, instead of being used to drive assimilation. These reversible changes in efficiency are known as photoprotection, and, if adequate, photoinhibitory damage to the water splitting PSII reaction center (photoinactivation) is avoided. Plants must constantly manage the trade-off between photoprotection and photoinactivation, while optimizing the photochemical efficiency of PSII.Some of the earliest systematic studies of photoinhibition were done by A. Ewart (1896) in Pfeffer’s laboratory in Leipzig 120 years ago (Figure 2). He examined the effects of excess light on the ability of chloroplasts in leaves to evolve O2. He detected this by examining the movement of O2-requiring bacteria towards photosynthetically active cells in leaf sections. Ewart is best remembered because he went on to translate three volumes of Pfeffer’s famous textbook (The Physiology of Plants) into English, and later became the first Professor of Plant Physiology in Australia (University of Melbourne, 1904).
Figure 2 Photoinhibitory printing of excerpts from the first page of Ewart's paper on assimilatory inhibition on a leaf of the shade plant Cissus antarctica. A microfilm negative of the text was paper clipped to the leaf which was exposed to full sunlight for an hour. Chlorophyll fluorescence was subsequently imaged with a special video camera. Those areas of the leaf exposed to strong light under the text show severely reduced fluorescence due to photoinhibition. The latent image persists for several weeks because these shade plants repair photoinhibitory damage only slowly (Osmond et al. 1999)
Modern research on photoinhibition of light reactions of photosynthesis was strongly influenced by the Dutch biophysicist Bessel Kok (1956), responsible for so many advances in photosynthetic research, and by the early field studies of CO2 exchange by Björkman and Holmgren (1963) in Sweden. Björkman’s sabbatical in Australia in 1971 stimulated renewed interest in photoinhibition, and with Australian and German collaborators, research in his Stanford laboratory has repeatedly changed the way people think in this field (Powles 1984; Demmig-Adams and Adams 1992). Australian research in photoinhibition continues to attract attention (Anderson et al. 1997; Matsubara et al. 2012; Jia et al. 2013). Although modern research techniques such as in vivo chlorophyll fluorescence are much more quantitative and field-portable, the questions being probed are remarkably similar to those studied by Ewart!
By and large, leaves on most plants cannot avoid harvesting light, but some have evolved with external features to forestall absorption of excess radiation. For example, leaves of desert plants often reflect a large part of incident light, the high reflectivity being due to hairs, salt crusts (as in Australian saltbushes such as Atriplex nummularia) or epidermal waxes (Robinson et al. ). Such features are effectively mechanisms for external photoprotection. In others such as Townsville stylo (Macroptilium atropurpureum) leaves demonstrate very effective light-avoiding responses when water stressed. Ludlow and Björkman (1984) showed that if leaves of Townsville stylo were restrained perpendicular to incident sunlight, high-temperature-dependent photoinhibition ensued. It was obvious that unrestrained movement in the field preserved green and functional stylo leaves under conditions that accelerated senescence of leaves on adjacent herbs and grasses. By way of contrast, in some species like the compass plant (Lactuca scariola) leaves actually track the sun’s movement to maximise light interception.
The best defence against photoinhibition is a photosynthetic apparatus organised to take advantage of bright light. Sun plants have high capacities for CO2 fixation and retain high photosynthetic efficiency at relatively high photon irradiance (see Figure 12.9, and Case study 12.1). Depending on photosynthetic pathway and environmental conditions, sun plants may also sustain high rates of non-assimilatory electron transport in carbon recycling during oxygenase photorespiration (Section 2.3) and in the water-water cycle (Mehler reaction; Asada 1999) in the absence of net CO2 fixation during stress. Sun plants are also well endowed with photoprotective mechanisms that facilitate a reversible downregulation of PSII efficiency and stimulate wastage of absorbed photons as heat in the antennae pigment–protein complexes, before transfer to the reaction centre of PSII. These processes, linked to the energetic status of thykaloids and the interconversion of xanthophyll pigments (Section 12.1.2), provide internal photoprotection for PSII reaction centres (Demmig-Adams and Adams 1992). Sun exposed leaves of some epiphytes with low photosynthetic capacity, such as dodder and mistletoes have two xanthophyll cycles and so seem doubly photoprotected (Matsubara et al. 2002). Collectively known as mechanisms of non-photochemical quenching (NPQ) of chlorophyll fluorescence, these mechanisms that protect against photoinactivation have become a remarkably active realm of plant structural biology (Osmond 2015).
For the most part, these antennae-based processes seem to accommodate photon excess in most natural environments. However, when photon excess is sustained, especially in combination with other stresses, photoinhibitory damage leading to photoinactivation of PSII reaction centres may follow. The site of damage in most cases seems to be the psbA gene product, the D1 protein which is the most rapidly turned over protein in chloroplasts. The D1 protein is also the binding site for the triazine family of herbicides and accounts for their lethal effects. Turnover of D1 is accelerated in bright light, and it is often described as the ‘suicide protein’ (Aro et al. 1993; Chow and Aro 2005). Sun plants have high rates of chloroplast protein synthesis, and are thus able to repair damage to the critical D1 protein of PSII reaction centres more readily than in shade plants (Section 1.2).
Shade plants, in which the photosynthetic apparatus is organised to take advantage of low light, are poorly endowed with all of the above processes. Some, such as the archetypical Australian shade plants Alocasia and South American Tradescantia (Park et al. 1996) show the dynamic internal light avoidance property of chloroplast movement to anticlinal cell walls in strong light. However, when exposed to sustained bright sunlight, in excess of that encountered during growth, shade-tolerant plants such as Alocasia at the margins of Queensland rainforests suffer photoinhibitory damage. Structural organisation of the photosynthetic apparatus reflects these biophysical and bio-chemical realities at all levels, and not surprisingly the very different granal structures of shade and sun plants have important implications for photoinhibitory damage (Anderson and Aro 1994; Matsubara et al. 2012). In the short term, shade plants accommodate bright light in sunflecks without photoinhibitory damage, and even exploit it for additional post-illumination CO2 exchange (Chazdon and Pearcy 1986; Pearcy and Way 2012).
Somewhat surprisingly, the inner canopy shade leaves of some trees, especially those of tropical origin, are also equipped with two xanthophyll cycles (García-Plazaola et al. 2007). For example, inner canopy avocado shade leaves engage the near universal, initially rapidly relaxing violaxanthin cycle during a strong sun fleck. If these leaves are exposed to prolonged sunlight (following a cyclone for example) the slowly relaxing lutein epoxide cycle “locks in” photoprotection. A traffic-light inspired holistic summary light intensity- and time-dependent interaction of these photoprotective processes is illustrated in Fig. 3 (Jia et al 2013). Photoacclimation to bright light, the successful long-term accommodation of photoinhibitory processes, is genetically limited in many species. Yet in avocado it is manifest as the retrofitting of old shade leaves to perform as sun leaves, with increased photosynthetic capacity and enhanced photoprotection involving two xanthophyll cycles.
Figure 3 The Yin and Yang of photoinhibition. Depending on genotype, irradiance and time the extent of photoinhibition (indicated by decline in ϕPSII) proceeds through engagement of internal mechanisms of photoprotection (indicated by the extent of NPQ). Rapidly reversible (seconds to minutes) NPQΔpH potentiates the more slowly reversible (minutes to hours) de-epoxidation of violaxanthin to antheraxanthin and zeaxanthin (NPQΔAZ) in light harvesting antennae of PSII in thylakoid membranes. In some plants, such as avocado, this photoprotection is augmented by a second de-epoxidation of lutein epoxide to lutein (NPQΔLAZ) that may persist for days. If still inadequate it will be followed by similarly slowly recoverable PSII reaction centre photoinactivation (NPQPI). (Reproduced from Jia et al. 2013. Plant Physiology 161, 836-852; Copyright American Society of Plant Biologists)
Although unicellular algae such as Chlamydomonas sp. have been widely used to research mechanisms of photoinhibitory damage (Förster et al. 2005), relatively much less is known of photoinhibition in either marine or freshwater environments. Under natural conditions, vertical movement of unicellular algae in water columns is an important determinant of photon exposure and photoinhibitory responses, which involve many of the same processes as in higher plants (Franklin et al. 2003). In addition, the ubiquitous marine macrophyte Ulva is susceptible to desiccation and high-temperature-dependent photoinhibitory damage in rock pools and estuaries when low tides occur at midday. The diversity of photosynthetic pigments among marine macrophytic algae suggests several alternative photoinhibitory mechanisms that are currently under investigation.
Clearly, photoinhibition is an integral and indispensable component of photosynthesis. The inefficiencies it produces in light utilisation are essential to the stability of the photosynthetic apparatus in organisms that depend on light for life, and especially in environments where they can do little to regulate the incoming flux of this basic resource. The costs of these inefficiencies remain difficult to estimate and the extent to which plant distribution in relation to sunlight is governed by photoinhibitory responses, remains controversial. Perhaps one of the most convincing examples is the interaction of bright light and low temperature which restricts re-establishment of eucalyptus seedlings to the shaded south side of parent trees on the Southern Highlands of New South Wales (Ball et al. 1991; Case study 14.1). New techniques for the remote sensing of chlorophyll fluorescence that monitor photosynthesis, photoprotection and photoinhibition offer exciting insights that will facilitate integration of these processes from leaves to canopies (Nichol et al. 2012) with the added prospect of ground truth for satellite observation of solar induced fluorescence at the landscape level. As with most aspects of plant biology today, genetic manipulations of many aspects of the above component photoprotective mechanisms are mooted to benefit plant productivity through mitigation of various aspects of photoinhibition (Ort et al. 2015).
This revision and update of Feature Essay 12.1 is dedicated to the memory of Professor Jan M Anderson (1932-2015) for her outstanding leadership and encouragement of Australian research in photosynthesis.
Anderson JM, Aro E-M (1994) Grana stacking and protection of photosystem II in thylakoid membranes of higher plant leaves under sustained high irradiance: an hypothesis. Photosyn Res 41: 315–326.
Anderson JM, Park Y-I, Chow WS (1997) Photoinhibition and photoprotection in nature. Physiol Plant 100: 214–223.
Aro E-M, Virgin I., Andersson B (1993) Photoinhibition of photosystem II. Inactivation, protein damage and turnover. Biochim Biophys Acta 1134, 113–134.
Asada K (1999) The water-water cycle in chloroplasts: scavenging of active oxygens and dissipation of excess photons. Annu Rev Plant Physiol Plant Mol Biol 50, 601-639.
Ball MC, Hodges VS, Laughlin GP (1991) Cold-induced photoinhibition limits regeneration of snow gum at treeline. Funct Ecol 5: 663–668.
Björkman O, Holmgren P (1963) Adaptability of the photosynthetic apparatus to light intensity in ecotypes from exposed and shaded habitats. Physiol Plant 16, 889–914.
Chazdon RL, Pearcy RW (1986) Photosynthetic responses to light variation in rainforest species. II Carbon gain and photosynthetic efficiency during lightflecks. Oecologia 69: 524–531.
Chow WS, Aro E-M (2005) Photoinactivation and mechanisms of recovery. In ‘Photosystem II. The light-driven water: plastoquinone oxidoreductase advances in photosynthesis and respiration’, Vol. eds TJ Wydrzynski, K Satoh, JA Freeman. 627–648, Springer, Dordrecht, The Netherlands)
Demmig-Adams B, Adams III WW (1992) Photoprotection and other responses of plants to high light stress. Annu Rev Plant Physiol Plant Mol Biol 43: 599–626.
Ewart AJ (1896) On assimilatory inhibition in plants. J Linnean Soc 31: 364-461.
Förster B, Osmond CB, Pogson BJ (2005) Improved survival of very high light and oxidative stress is conferred by spontaneous gain-of-function mutations in Chlamydomonas. Biochim Biophys Acta (Bioenerg) 1709, 45-57.
Franklin LA, Osmond CB, Larkum AWD (2003) Photoinhibition, UV-B and algal photosynthesis. In Photosynthesis in Algae. Advances in Photosynthesis and Respiration, Vol. eds AWD Larkum, SE Douglas, J A Raven. 351-384, Kluwer, Dordrecht.
García-Plazoala J-I, Matsubara S, Osmond CB (2007) The lutein epoxide cycle in higher plants: its relationship to other xanthophyll cycles and possible functions. Funct Plant Biol 34, 754-779.
Jia HS, Förster B, Chow WS et al. (2013) Decreased photochemical efficiency of Photosystem II following sunlight exposure of shade-grown leaves of avocado (Persea americana Mill.): because of, or in spite of, two kinetically distinct xanthophyll cycles? Plant Physiol 161: 836-852.
Kok B (1956) On the inhibition of photosynthesis by intense light. Biochim Biophys Acta 21: 234–244.
Ludlow MM, Björkman O (1984. Paraheliotropic leaf movement in Siratro as a protective mechanism against drought-induced damage to primary photosynthetic reactions: damage by excessive light and heat. Planta 161: 505–518.
Matsubara S, Gilmore AM, Ball MC et al. (2002) Sustained down regulation of photosystem II in mistletoes during winter depression of photosynthesis. Funct Plant Biol 29: 1157-1169.
Matsubara S, Förster B, Waterman M et al. (2012) From ecophysiology to phenomics: some implications of photoprotection and shade-sun acclimation in situ for dynamics of thylakoids in vitro. Phil Trans Royal Soc London B 367: 3503-3514.
Nichol CJ, Pieruschka R, Takayama K et al. (2012) Canopy conundrums: building on the Biosphere 2 experience to scale measurements of inner and outer canopy photoprotection from the leaf to the landscape. Funct Plant Biol 39: 1-24.
Ort D R et al. (2015) Redesigning photosynthesis to sustainably meet global food and bioenergy demand. Proc Nat Acad Sci USA 112: 8529-8536.
Osmond B, Schwartz O, Gunning B (1999) Photoinhibitory printing on leaves, visualised by chlorophyll fluorescence imaging and confocal microscopy, is due to diminished fluorescence from grana. Aust J Plant Physiol 26: 717-724.
Osmond B (2015) An ecophysiologist’s apology. Book Review: Non-photochemical Quenching and Energy Dissipation in Plants, Algae and Cyanobacteria, Advances in Photosynthesis and Respiration, Vol 40. eds B. Demmig-Adams, G. Garab, W.W. Adams III and Govindjee (eds) (2014), Springer, Dordrecht. Photosyn Res 124:127-130
Park Y I, Chow WS, Anderson JM (1996) Chloroplast movement in the shade plant Tradescantia albiflora helps protect photosystem II against light stress. Plant Physiol 111: 867-875.
Pearcy RW, Way DA (2012) Two decades of sunfleck research: looking back to move forward. Tree Physiol 32 1059-1061.
Powles SB (1984) Photoinhibition of photosynthesis by visible light. Annu Rev Plant Physiol 35: 15-44.
Robinson SA, Lovelock CE, Osmond CB (1993) Wax as a mechanism for protection against photoinhibition - a study of Cotyledon orbiculata. Bot Acta 106: 307-312.
Solar radiation is linked to agricultural productivity via biomass production and allocation to harvested parts such as grains and fruit. Radiation in this context is in relation to canopy photosynthesis. Biomass is derived from photosynthesis, but is less than the total carbon that is assimilated due to a large respiratory loss by the plant (see Case study 12.1). Carbon lost via respiration is, however, a fairly constant proportion of photosynthesis, and thus variation in canopy photosynthesis is sufficient to account for variation in biomass production.
How then does interception of photosynthetically active radiation (PAR) or photon irradiance affect biomass production and allocation to crop yield? Three steps are considered in this section: (1) variation in the incident PAR during crop growth, (2) interception of PAR by a crop canopy and (3) efficiency of PAR conversion into biomass and yield.
Crop yield commonly depends on the total amount of light intercepted, particularly when crop growth is not limited by other factors such as nutrient or water deficiency or temperature extremes. One example highlighting the importance of solar radiation for crop yield comes from a comparison of rice crops in Australia with those grown in tropical areas.
Rice in Australia is grown almost exclusively in southern New South Wales during dry summer months (November–March). Crops are fully irrigated and well fertilised and yield around 9 t ha–1. This high yield is associated with high incident solar energy (commonly 10–15 MJ m–2 d–1 PAR) during the long growing season. In tropical Asian countries, rice is commonly grown under cloudy conditions during the wet season (June–November). Yield is lower (4–5 t ha–1) even with high nutrient inputs, because of a shorter growing season and lower solar energy (often around 8–10 MJ m–2 d–1 PAR). Experiments with shading treatments have shown that growth and yield of rice and many other agricultural crops, are reduced by decreased solar radiation.
Interception of light by a crop canopy is strongly related to total or canopy leaf area. A crop will thus intercept more light and hence grow faster if it develops the canopy leaf area rapidly. This principle applies to both annual crops, which are usually planted at the beginning of a growing season and to perennial crops, which resume growth after a dormant season. Leaf area development of sugar cane, for example, is generally slower in the year of planting compared with a subsequent ratooned crop, where canopy regrowth is enhanced by stored photoassimilates. By analogy with early canopy expansion, retention of green leaves late in a growing season also extends light interception and enhances storage of photoassimilates. This is also true for perennial crops. For some deciduous horticultural crops, leaf area expansion is also rapid because of preformed primordia which emerge rapidly and comprise the largest leaves (Greer 1996).
The leaf area index (LAI) is the ratio of total projected leaf area (one side only) per unit ground area, and is widely used to characterise the canopy light climate. A canopy where LAI equals 1 has a leaf area equal to the soil surface area on which it grows. This does not mean all light is intercepted, because some leaves overlap, leaving gaps. Moreover, not all leaves are positioned at right angles to the incident radiation. A crop under favourable growing conditions increases LAI rapidly during early development to a maximum of 3 to 7.
An example of LAI development of three tropical cereal crops grown under well-watered conditions in South East Queensland is given in Figure 12.19. Sorghum showed a more rapid increase in LAI than did maize, largely because of a higher sowing density (33 v. 5.6 plants m–2). A late maturing rice crop showed slowest leaf area development during early stages of growth, but the maximum LAI was none the less higher for rice than for maize. As a general rule, maximum LAI is achieved just prior to flowering in cereal crops. By that stage, growing points are differentiating floral rather than leaf primordia, and initiation of new leaves has ceased.
Some cereal crops lose leaves and the LAI declines during grain filling as crops mature. Differences in LAI development among the three crops (Figure 12.20a) are evident in light interception by the respective canopies (Figure 12.19b); interception prior to 60 d was highest in sorghum and lowest in rice. However, in all three crops, canopy light interception increased rapidly during early stages of growth. Incident radiation was almost completely intercepted once a high LAI had been achieved.
Despite wide variation in crop phenology, sunlight interception and LAI maintain a tight curvilinear relationship (Figure 12.19c). Thus, interception increases sharply with increases in LAI to about 90% once LAI exceeds 4, and approaches an asymptote at higher LAI, see also Figure 12.20 from Khurana and McLaren (1982). In this research on potato, numerous treatments were imposed, involving different storage of seeds at low temperatures including apically and multisprouted seed treated at 4 and 12 °C and then at 8 °C before planting. In Treatment1, the trial included unsprouted seed stored at 4 °C, in Treatment 2, the trial included seed stored in the dark and in Treatment 3, two additional sprouting treatments were mixed alternately along the row. Such a relationship between LAI and light interception applies to many crops, and emphasises (1) the importance of a rapid increase in LAI during early stages of growth, and (2) a requirement for only moderate LAI to achieve effective light interception. Indeed, excessive leaf area development can be counter-productive, because reproductive development, and hence economic yield, may be reduced due to self-shading and resource allocation to leaf production (such as for fruit trees and grapevines, Section 12.4).
Figure 12.20 The proportion of incident radiation intercepted by potato canopies as a function of leaf area index. The different symbols indicate agronomic treatments over two growing seasons. In 1979: □ cv. Record, ○ cv. Pentland Crown. In 1980: cv. Pentland Crown with three treatments (see text): ●Treatment 1, ▲Treatment 2, ■ Treatment 3. (Based on Khurana and McLaren 1982)
The time-course of light interception during crop growth can be manipulated to some extent by farmers. For example, seeding rate is an important management option which affects interception and subsequent crop growth and yield. A high seeding rate would produce a high plant population density and a high LAI at crop establishment. This hastens canopy interception and hence biomass production would be promoted. Any advantage of a high plant density may, however, disappear with time during crop growth, because radiation interception of a medium plant density may eventually catch up with that of the high density (Figure 12.21).
In this case, density 3 (35 plants m–2) was sufficient for radiation interception and plant dry matter production. If plant density is very low, shown as density 1 (1.4 plants m–2) or density 2 (7 plants m–2) in Figure 12.21, LAI never exceeded 2 and final biomass at harvest was much smaller than values returned from higher densities. Solar radiation was not fully intercepted and hence wasted at low planting density, and potential yield (dry mass produced per unit area) was never realised.
As solar radiation penetrates a crop canopy, PAR is intercepted by leaves and photon irradiance commonly declines exponentially with cumulative leaf area (i.e. depth in Figure 12.23), according to the exponential relationship:
\[ I = I_0 e^{-kL} \tag{12.1} \]
where \(I\) is horizontal photon irradiance within a canopy, \(I_0\) is horizontal photon irradiance above that canopy, \(L\) is LAI from the top of the canopy to the point where \(I\) is determined, and \(k\) is an extinction coefficient (a more explicit formulation for PAR attenuation through a forest canopy is given in the next section).
Large \(k\) values imply that photon irradiance decreases rapidly with depth, whereas a canopy with a small \(k\) would allow solar radiation to penetrate deeply, for a similar leaf area profile. Variation in \(k\) value is commonly associated with leaf angle. Canopies with more horizontal leaves, such as sun-flower or cotton, have large \(k\) values, often 0.7–1.0, whereas those with more erect leaves, such as barley and sugar cane, have small values, often 0.3–0.6.Irrespective of the canopy extinction, there is a strong relationship between the light intercepted by the canopy over the growing season and the total dry matter produced for a number of crops (Fig. 12. 22). Thus, it is imperative on growing crops to manage the LAI to achieve maximum light interception from the early part of the season to maximise production of dry matter.
Canopy structure, and particularly the spatial distribution of leaf angles, has an important bearing on the canopy light climate and energy conversion. Large leaf angles, with leaves close to vertical, ensure good light penetration when solar angle is high, and a high proportion of leaves receive similar photon irradiances. An even distribution of light at leaf surfaces is advantageous for canopy photosynthesis and improves light use efficiency over canopies where upper horizontal leaves intercept most solar radiation and lower leaves experience greatly attenuated levels. Small and erect leaves, particularly in top canopy layers, are thus a key feature of an ideal plant type, or ‘ideotype’ for high-density cropping (Figure 12.23).
Canopy radiation climate is especially complex in mixed crops and pastures where species with contrasting forms grow together. In grass–legume pastures, grass is generally taller than the legume component and is better placed to intercept incident radiation. Legumes then exist in permanent shade. Height is, therefore, an important determinant of light interception within a mixed sward, and thus species composition. In such mixed swards, management options such as nitrogen fertiliser application, grazing time or cutting frequency all affect the relative height and hence radiation interception by component species. High-nitrogen fertiliser tends to favour grass, while clover may become dominant under nitrogen-limiting conditions.
Light profiles within a pasture are, therefore, affected by LAI profiles of component species (Figure 12.24), and a clover-rich sward with more horizontal leaves (N0, no added nitrogen) shows stronger attenuation of sunlight than a grass-rich sward with a preponderance of vertical leaves (N225, nitrogen added). In common with monocultures, pasture productivity is enhanced by a species balance that ensures even distribution of sunlight within a mixed community.
Tree canopies cast shadows, especially on clear days, indicating absorption of radiant energy. Averaged over space and time, the photosynthetically active component of that energy is efficiently employed and drives growth. Acquisition of energy and carbon by plants is thus determined by total leaf area, leaf surface distribution within the canopy and photosynthetic capacity of individual leaves. Productivity will ultimately depend on distribution of photosynthetic performance throughout the canopy as a whole, which in turn, is determined by the photosynthetic capacity of individual leaves and the distribution of sunlight.
Plant canopies are structurally diverse because of unique spatial patterns that different species adopt for intercepting light and the diversity of plant species which occupies a natural community. For example, there is considerable penetration of sunlight through the canopy of a dry eucalypt forest. Conversely in dense rainforest or in a radiata pine plantation, only sunflecks reach the ground. A considered glance from ground to tree top reveals why these dissimilarities occur (Figure 12.25).
Experiments were in progress at this site in Cameroon on microclimate responses to forest management. Trees had been clear felled mechanically, clear felled manually or selectively cleared. Growth rate of newly planted saplings was measured in these plots and compared to growth in undisturbed plots. Hemispherical photographs were used to calculate change in canopy cover and solar radiation load on different plots (shown here). Hemispherical photographs can also be used to calculate potential contribution from sunflecks by plotting the sun's path across a photograph.
The first reason for such dissimilarities is based on canopy density or the quantity of leaf area per unit canopy volume. This index is substantially less for a dry eucalypt forest than that for a rainforest or pine forest. The second reason relates to the display of the foliage. Adult leaves of eucalypts are typically pendulous, allowing much of the incident light and energy to pass uninterrupted through the canopy and to reach the ground. Conversely, in a diverse rainforest, many species display their leaves at shallower angles to the horizontal, thereby absorbing a larger proportion of incident radiation and preventing much of the incident light and energy being transmitted to the ground.
In a complex system like a rainforest, the canopy is arranged in horizontal layers, the distribution of leaf area with height being associated with the development in space and time of the diversity of species. However, even in monocultures it is convenient to consider the canopy as being horizontally uniform and the level of radiation constant in any layer. Even here, attenuation of light through the canopy is complicated by changing availability, quality and direction of incident light and it is necessary to make some simplifying assumptions when calculating the proportion of light that is intercepted.
The Beer–Lambert Law, which describes absorption of light by plant pigments in solution, provides a simple approach which has been applied widely to a range of canopies. This function demonstrates that the absorption of light will be more or less decline exponentially with increasing intercepting area down through the canopy. Absorption of sunlight by photosynthesis occurs within a well-defined spectral band (400–700 nm) and matches a peak in energy distribution across the wavelength spectrum of sunlight transmitted to the earth’s surface through our atmospheric window (as shown in Figure 12.1 at the start of the chapter).
Sunlight in this waveband can be represented as either a quantum flux or a radiant energy flux. Quantum flux, or more explicitly, photosynthetic photon flux density (PPFD, PAR), is simplified here to ‘photon irradiance’ (\(Q\)) and has units of µmol quanta m–2 s–1 (‘µmol quanta’ rather than ‘µmol photons’ because quantum energy derived from photons drives photosynthesis). For the sake of making a clear distinction, radiant energy flux is simplified to ‘irradiance’. In the present example, irradiance coincides with photosynthetically active radiation (PAR) and is expressed as joules (J) per square metre per unit time. Depending on the application, time can span seconds, days or years, and is then coupled with either joules, megajoules (MJ) or gigajoules (GJ).
On clear days, PAR represents about half of the total shortwave (solar) radiation or radiant energy flux \(I\) (expressed as J m–2 s–1) incident on a canopy, and is totally responsible for photosynthesis. If changes in the spectral distribution of energy as it passes through the canopy are ignored, \(I\) and PPFD can be used interchangeably in the analysis below. In practice, PPFD is attenuated more rapidly than \(I\) (that is, there is a proportionally larger change in PAR than total solar radiation (\(I\)) in moving from top to bottom of the canopy) because leaves are relatively transparent to the near-infrared part of the solar beam.
Application of the Beer–Lambert Law shows that at any level of cumulative area \(F\) within the canopy, the rate of change of photon irradiance, \(Q\), within the canopy is given by:
\[ \mathrm{d} Q / \mathrm{d} F = -kQ_F \tag{12.2} \]
where \(k\) is the extinction or foliar absorption coefficient, a dimensionless parameter. \(k\) measures the fraction of incident photons absorbed by a unit of leaf area or conversely the fraction of leaf area projected onto the horizontal from the direction of the incident beam. For many species, foliage in the vertical plane is distributed approximately symmetrically about the midpoint of the canopy and most absorption of light will occur in the middle of the canopy. After integration, \(Q_F\) at any level \(F\) is given by:
\[ Q_F = Q_0 e^{–kF} \tag{12.3} \]
where \(Q_0\) is the PAR incident at the top of the canopy. At the base of the canopy, \(F\) is equal to the leaf area index (LAI), a dimensionless number which expresses total projected leaf area of the canopy as a ratio of the ground area over which it is displayed. Thus the level of interceptance is an exponential function of the product \(kF\). If a value of 0.5 is assigned to \(k\), then 95% light interception occurs at \(kF = 3\) which is equivalent to an LAI of 6 m2 leaf area m–2 ground area. Maximum values of LAI vary with species, site, stress and season.
In practice, \(k\) is not a constant value for any canopy and varies with solar elevation, the ratio of direct to diffuse beam irradiance and any changes in canopy structure or leaf inclination and orientation which occur seasonally or in response to the movement of leaves (e.g. heliotropism). For the majority of canopies, \(k\) varies from 0.3 to 1.3. Canopies with erectophile leaves (e.g. grasses) and high leaf angles to the horizontal or with a clumped distribution have a lower \(k\) and intercept less light per unit of foliage compared to canopies with planophile leaves with a higher \(k\) (e.g. clovers) and low leaf angles or a regular distribution. The cumulative leaf area required to intercept 95% of the radiation incident at the top of the canopy will be greater for canopies dominated by erectophile leaves or having a clumped distribution.
In many species and plant communities, leaf inclination may change from erectophile at the top of the canopy to planophile at the bottom. This allows more even distribution and interception of light and reduces the proportion of leaves which is exposed at the top of a canopy to levels of light which are saturating for photosynthesis and, conversely, reduces the proportion of leaves at the bottom of a canopy which is exposed to levels below the light-compensation point for photosynthesis. For a canopy with leaves distributed randomly with respect to orientation and inclination, \(k\) is approximately 0.5 (Monteith and Unsworth 1990) and this value is commonly assigned to \(k\) in the literature.
Photosynthesis is driven by the fraction of radiation intercepted by the canopy and gross photosynthetic production (\(A_g\)), a measure of the total amount of CO2 fixed in photosynthesis, can be expressed as:
\[ A_g = A_0 [1 - exp(-kS_{a}W_{l})] \tag{12.4} \]
\(A_0\) is the gross photosynthetic production at full light interception and \(S_{a}W_{l}\) expresses LAI as the product of specific leaf area (\(S_{a}\), the ratio of leaf area:leaf dry mass) and dry mass of leaf organic matter (\(W_{l}\), often approximated as leaf dry mass).
As implied by Equation 12.4, there is a proportional relationship between production of dry mass and interception of radiation (see Fig. 12.22), while LAI is a major determinant of photosynthetic production. The slope of this relationship is a measure of the conversion efficiency (\( \varepsilon \)) of light (photon irradiance if based on PAR) or irradiance (\(I\) if based on shortwave radiation) to dry mass. \( \varepsilon \) has units of g MJ–1 and values based on photon irradiance are approximately twice those based on \(I\). \( \epsilon \) can be considered to be the canopy-scale equivalent of \( \varphi \), the quantum yield of individual leaves. Values of \( \varepsilon \) based on absorbed radiation are net of any light or radiation that is reflected upward from the direction in which the incident value is measured.
This proportional relationship was first clearly defined in the above terms for the seasonal growth of temperate agricultural and horticultural crops in Britain (Monteith 1977, Fig. 12.22). It has since been shown to hold for a range of vegetation types and environments. Proportionality occurs because photosynthesis by most leaves in a canopy tends to be light limited. Consequently any increase in light intercepted or absorbed results in an increase in dry mass production. As crops grow from establishment or plant communities develop from a state of initial colonisation to maturity, LAI increases, and a greater leaf surface results in greater levels of light interception and rates of growth.
Figure 12.26 Above-ground dry mass production and intercepted radiation for eucalypts in plantations in Tasmania and Victoria show a linear relationship. Closed symbols refer to different species or provenances of the same species at Tasmanian sites. Open symbols refer to Eucalyptus globulus growing in Victoria. (Original data C.L. Beadle and G. Inions)
As predicted by theory, linear relationships between above-ground dry mass production and intercepted radiation have been observed for eucalypts in plantation forests in southeast Australia: \( \varepsilon \) was around 0.45 g MJ–1 (based on \(I\), Figure 12.26). Conversion efficiency was independent of species and provenance within species, and for one species, Eucalyptus globulus, was independent of site. Differences in growth rate between species, in this case during the early phase of forest growth, can be entirely a function of more rapid development of LAI in one species compared to another.
Comparative analyses of \( \varepsilon \) for different vegetation types are commonly based on above-ground dry mass (\(W_{a}\)) because of lack of information on below-ground biomass (\(W_{b}\)). \( \varepsilon \) based on \(W_{a}\) will be less than that based on \( W_{a} + W_{b} \) by the ratio \(W_{a} : (W_{a} + W_{b}) \). Partitioning of dry mass to roots may be substantially higher on a resource-poor compared to a resource-rich site. Consequently, a comparison of \( \varepsilon \) between sites based on \(W_{a}\) rather than \( W_{a} + W_{b} \) would lead to a relative underestimate of the efficiency of conversion of light to dry mass on poorer sites. Similarly, stress in response to soil water deficit, high vapour pressure deficit (leading to stomatal closure) or extremes of temperature will reduce \( \varepsilon \) and may also change the partitioning of dry mass. Reductions in \( \varepsilon \) occur in response to stomatal closure or to stresses of sufficient severity to reduce the quantum yield of photosynthesis. In effect, plants reduce photosynthesis by redirecting absorbed energy away from photochemistry and into photoprotective pathways which disperse absorbed energy as heat. \( \varepsilon \) thus embodies the photosynthetic history of a crop over a given interval and integrates the effects of all environmental variables on photosynthetic utilisation of absorbed radiation.
In summary, forest canopies consist of multiple layers. A logarithmic gradient of sunlight availability exists from upper to lower layers, and leaf properties adjust accordingly.
Canopy photosynthesis depends on distribution of sunlight over individual foliage elements. Because light is unevenly distributed within canopies, leaf photosynthetic rates vary spatially. Photosynthesis also varies temporally because of fluctuating environmental conditions. This fine-scale variability in light distribution and photosynthesis has been successfully described by detailed simulation models of canopy processes. An alternative modelling approach is to ignore fine-scale variability and to focus instead on canopy-scale relationships. That approach was pioneered by Monteith (1977) examining the relationship between dry matter production and absorbed photosynthetically active radiation (APAR) for canopies of four crops (apples, barley, potatoes and sugar beet) under ideal growing conditions in Britain (see Figure 12.22). Monteith discovered that these relationships were linear and that their slopes, the so-called light utilisation coefficient (\(\varepsilon\)), were similar for all four species (\(\varepsilon\) ~ 2.8 g dry mass MJ–1 PAR).
Monteith regarded the value for \(\varepsilon\) of 2.8 g dry mass MJ–1 as an upper limit to growth efficiency and used it to estimate potential arable crop production of Britain. He also observed that field-grown arable crops usually have values of \(\varepsilon\) well below this upper limit.
Monteith’s study was followed by similar studies on other crops (e.g. Muchow and Davis 1988) and trees (Landsberg et al. 1996). This work has tended to confirm the relationship’s linearity, but has revealed that the slope \(\varepsilon\) varies considerably between species, and is greatly reduced when growing conditions are suboptimal. For tree stands, values of \(\varepsilon\) are usually evaluated as above-ground dry matter yield per unit APAR. Published values range from 0.2 g MJ–1 for older tropical forest stands to 2.8 g MJ–1 for young pot-grown Salix and Populus stands (Landsberg et al. 1996). Values of \(\varepsilon\) are given in Table 2 for four pine species growing at five experimental sites with contrasting environments in Australia, New Zealand, the USA and Sweden. Across these contrasting environments, one boreal, one subtropical and three temperate, above-ground productivity ranged from 0.39 to 3.2 kg m–2 year–1, wood production from 0.22 to 2.5 kg m–2 year–1, and values of \(\varepsilon\), derived from measured above-ground production and simulated APAR, from 0.27 to 1.4 g MJ–1.
For the pine stands considered in Table 2, sufficient data are available to analyse causes of variation in \(\varepsilon\). A useful starting point for that analysis is the biochemical upper limit to \(\varepsilon\). That limit, determined by the quantum requirement of photosynthesis, is characterised by the quantum yield of photosynthesis, which for C3 plants (modified for the spectral composition of light and photorespiratory carbon loss) is conservatively 0.06 mole CO2 per mole absorbed quanta, corresponding to an energy conversion efficiency of 6%. That efficiency can be converted to an equivalent light utilisation coefficient if we know the energy content of incident light, a function of its spectral composition. Assuming an energy content of 0.22 MJ mol–1 and assuming the carbon content of biomass is 0.45 gives an equivalent light utilisation coefficient of approximately 7.3 g dry mass MJ–1.
There are several reasons why this biochemical upper limit is not attained in nature. One reason is that leaf photosynthetic efficiency declines as quantum flux increases; according to models of canopy photosynthesis, this light saturation of photosynthesis leads to a reduction of 50–60% in photosynthetic carbon gain (i.e. a loss factor of 0.45). Values of \(\varepsilon\) are further reduced because of respiratory losses associated with the maintenance of living tissue and the growth of new tissue; gas exchange measurements in pine forests show that this process leads to a reduction of 40–60% for closed-canopy forests (loss factor = 0.5) (Figure 2). Another reason for the reduction of \(\varepsilon\) is carbon allocation to root growth, approximately 15–25% for highly fertile stands (loss factor = 0.8). The combined effect of light saturation, respiratory losses and below-ground allocation is to reduce \(\varepsilon\) to 7.3 x 0.45 x 0.5 x 0.8 = 1.3 g MJ–1, a value which is similar to that estimated from measured growth of Pinus radiata in highly productive New Zealand and Canberra stands (Table 2).
Further reductions of \(\varepsilon\) occur if stands are nutrient, water or temperature limited, which is the case for the other four stands in Table 2, or if trees suffer disease or insect damage. If we regard an \(\varepsilon\) value of 1.3 g MJ–1 as the maximum achieved by fertile, well-watered, closed-canopy pine stands, then the remaining reductions of \(\varepsilon\) at the stands in Canberra (control), Florida, Wisconsin and Sweden are approximately 50, 35, 80 and 60%, respectively. Stands experiencing temperature extremes or water stress can have shortened effective growing seasons, which affect \(\varepsilon\) because radiation intercepted outside the active growing season is less efficiently utilised. Estimated effective growing season lengths for the five sites are given in Table 2. The growing season is shortest at the Wisconsin site, which experiences both harsh winters and hot, dry summers (\(\varepsilon\) = 0.27 g MJ–1), followed by the Swedish site, which experiences extremely cold winters (\(\varepsilon\) = 0.56 g MJ–1). For the control stand at Canberra, the effective growing season is reduced by summer droughts which cause stomata to close and photosynthesis to cease almost entirely during periods of extreme water stress (\(\varepsilon\) = 0.66 g MJ–1); note that above-ground production by this stand is less than half that of an irrigated + fertilised stand at the same site, whereas its value of APAR is only 15% lower.
Site nutrition can also affect \(\varepsilon\) by altering either total carbon gain, or the proportion of carbon allocated to root growth; for example, soil fertility is poor at the Florida, Sweden and Canberra (control) sites, all of which have low \(\varepsilon\) values. The Canberra experiment is an interesting illustration of the effect of nutrition on below-ground carbon allocation (Figure 2); fine-root production is lowest on the irrigated + fertilised plot, 8% of net primary production (NPP) compared with 20% on the control and irrigated stands (Ryan et al. 1996).
Several conclusions can be drawn from this analysis of light utilisation. First, the above \(\varepsilon\) model can explain observed productivities of fertile, well-watered pine stands as the estimated biochemical limit minus unavoidable losses associated with light saturation of photosynthesis, respiration and below-ground allocation. For these highly productive forests, the largest single loss factor for \(\varepsilon\) is associated with light saturation of photosynthesis (loss factor = 0.45). The magnitude of that reduction depends on both leaf physiology, particularly light-saturated rates of photosynthesis, and the within-canopy light environment, which in turn is a function of cloudiness and canopy structural properties such as shoot structure and crown architecture.
A second conclusion from the data in Table 2 is that in four of the six stands, extreme weather conditions reduce the effective length of the growing season, and that this reduction is critical in explaining differences in \(\varepsilon\) among forest stands. Shortened growing seasons appear to be the primary reason why \(\varepsilon\) is low for the Wisconsin, Swedish and Canberra (control) stands. For species experiencing cold winters (Wisconsin and Sweden), it is important to understand how temperature and daylength affect leaf phenology, especially rates of leaf growth and photosynthesis as trees emerge from winter dormancy. The low value of \(\varepsilon\) for Pinus radiata at the control stand in Canberra is due largely to water stress, with low annual rainfall coupled with high transpiration rates in summer months, leading to rapid depletion of soil water reserves and a shortened effective growing season.
A sidelight to the above discussion is the observation that NPP is proportional to APAR at the canopy scale (Section 12.3.2, Figure 12.26), although leaf photosynthetic rates saturate at high quantum flux. This puzzling observation appears to be largely explained by the different time scales used in measurements of NPP and photosynthesis; NPP is usually measured over a growing season whereas photosynthesis is measured over periods of seconds or minutes. When canopy models are used to evaluate short-term (e.g. daily) NPP, saturation is found at high quantum flux (Medlyn 1996; Sands 1996). However, simulated annual values of \(\varepsilon\) are relatively constant because annual incident PAR varies little from year to year. Other studies have proposed that \(\varepsilon\) is constant because of compensatory effects of leaf area index, incident PAR and leaf nitrogen content (e.g. Sands 1996). Either way, prediction and observation of NPP have proved congruent across a wide range of genotype × environment combinations, and confirm the robustness of process-based simulation models.
Gower ST, Gholz HL, Nakane K, Baldwin VC (1994) Production and carbon allocation patterns of pine forests. In HL Gholz, S Linder, RE McMurtrie, eds. Environmental Constraints on the Structure and Productivity of Pine Forest Ecosystems: A Comparative Analysis. Ecological Bulletins (Copenhagen), Vol. 43. Munksgaard International Booksellers: Copenhagen, pp 115-133
Landsberg JJ, Prince SD, Jarvis PG et al. (1996) Energy conversion and use in forests: an analysis of forest production in terms of radiation utilization efficiency (ε). In HL Gholz, K Nakane, H Shimoda, eds. The Use of Remote Sensing in the Modeling of Forest Productivity. Kluwer, Dordrecht, pp 273–298
McMurtrie RE, Gholz HL, Linder S et al. (1994) Climatic factors controlling the productivity of pine stands: a model-based analysis. In Ecological Bulletins (Copenhagen), Vol. 43. As above, pp 173–188
Medlyn BE (1996) Interactive effects of atmospheric carbon dioxide and leaf nitrogen concentration on canopy light use efficiency: a modeling analysis. Tree Physiol 16: 201–209
Monteith JL (1977) Climate and the efficiency of crop production in Britain. Phil Trans Royal Soc London, Series B 281: 277–294
Muchow RC, Davis R (1988) Effect of nitrogen supply on the comparative productivity of maize and sorghum in a semi-arid tropical environment. II. Radiation interception and biomass. Field Crops Res 18: 31–43
Ryan MG, Hubbard RM, Pongracic S, Raison RJ, McMurtrie RE (1996) Foliage, fine-root, woody-tissue and stand respiration in Pinus radiata in relation to nitrogen status. Tree Physiol 16: 333–343
Sands PJ (1996). Modelling canopy production. III. Canopy light-utilisation efficiency and its sensitivity to physiological and environmental variables. Aust J Plant Physiol 23: 103–114
An upper limit on dry matter production by crops is set by the amount of visible radiation (400–700 nm) intercepted (Monteith 1977). Horticultural crops, however, rarely achieve 100% interception of sunlight because physical access is required year round for routine management operations such as spraying, pruning and picking. This is especially so for perennial fruit crops and vines, but is also the case for many annual greenhouse and vegetable crops that are repeatedly hand harvested. With a closed agronomic canopy such as a field of wheat, light interception is largely determined by LAI. With discontinuous canopies such as an apple orchard or a vineyard, a fixed LAI can intercept different amounts of light depending upon the display in space of the discrete canopy units, namely individual trees or vines. (LAI for discontinuous canopies is calculated from the total area of leaves on the plant divided by the area allocated to the plant.)
Light interception of horticultural crops can be altered by changing spacing, tree height, tree shape and/or row orientation. An illustration of this is given in Table 12.1 using a simulation model of light interception (Palmer 1989). The model calculated light interception over a whole day and allowed for change in incident radiation, sun angle (both elevation and azimuth) and sky light. The model was used to calculate light interception under sunny conditions by apple trees arranged in continuous rows, of truncated conical cross-section, in midsummer at Riwaka, Nelson, New Zealand. For a constant LAI, light interception increased by increasing hedge height, closer row spacings and planting in a north–south rather than an east–west orientation (Table 12.1). Light interception varied by a factor of two, from 27% by short wide-spaced hedges in east–west (EW) rows to 55% by tall close spacings in north–south (NS) rows. Differences due to row orientation will be smaller in cloudy regions where more of the incoming light is diffuse.
In Table 12.1, the density of leaves within the tree volume changes with height and row spacing to maintain a constant LAI. Differences in light interception would be much larger if leaf area density rather than LAI was maintained at a constant. Nevertheless, data given in Table 12.1 for discontinuous canopies show that the relationship between LAI and light interception depends on the physical arrangement of foliage in space. Calculations for Table 12.1 assume an extinction coefficient appropriate for apples. Light interception would have to be recalculated for other crops such as peach and cherry that have lower extinction coefficients (Flore 1994).
As discussed in Section 12.3, the relationship between light interception (\(F\)) and LAI of a continuous canopy follows the Beer–Lambert Law, and can be approximated by Equation 12.5. Again, \(k\) (the extinction coefficient) is dependent upon leaf angle distribution, leaf transmission to sunlight and whether the foliage is random, regular or clumped. \(k\) varies between about 0.3 for erect foliage and 1.0 for canopies comprising horizontally disposed leaves. In general terms,
\[ F = 1 - e^{–k\mathrm{LAI}} \tag{12.5} \]
Unlike closed canopy forests or a continuous crop cover, sunlight interception by discontinuous canopies consists of two parts, a fraction determined by the overall form and extent of the canopy (\(F_\mathrm{max}\)) and a leaf area part that determines how close a particular orchard approaches the maximum interception attainable. \(F_\mathrm{max}\) would be reached if LAI was infinite. Accordingly, Equation 12.5 can be rewritten for discontinuous canopies as Equation 12.6, where \(L' = \mathrm{LAI}/F_\mathrm{max}\) (Jackson and Palmer 1979).
\[ F = F_\mathrm{max} (1 - e^{–kL'}) \tag{12.6} \]
This relationship for a discontinuous canopy contrasts with that for the continuous canopy (Equation 12.5) where an infinite LAI would cause complete exclusion of light. When light interception by two of the orchards in Table 12.1 is plotted as a function of LAI (Figure 12.29) and compared with a continuous canopy with the same extinction coefficient, light interception by either orchard approached an asymptote well short of infinite LAI.
Orchards were either a continuous canopy (\(F_\mathrm{max}\) = 100%), hedgerows 2 m tall, with 3 m row spacing, 1.5 m thick at the base and 0.5 m at the top (\(F_\mathrm{max}\) = 74%) or hedgerows 1 m tall, with 5 m row spacing, 1.5 m thick at the base and 0.5 m at the top (\(F_\mathrm{max}\) = 39%). Note that light interception depended on both LAI and \(F_\mathrm{max}\), so that the same light interception can be achieved with a very different LAI or the same LAI can be rearranged to intercept a different amount of sunlight.
Light interception by discontinuous orchard canopies can, therefore, be increased by increasing LAI, but a far more effective strategy is to increase canopy extent. Put another way, it is better to use orchard design to increase \(F_\mathrm{max}\) and thereby intercept sunlight that would otherwise fall into clear alleyways, rather than intercept sunlight that had a good chance of being absorbed by other foliage. Knowing the physical dimensions of the canopy, \(F_\mathrm{max}\) can be calculated from a mathematical model. \(F_\mathrm{max}\) complex shapes can be determined from CAD graphics.
As for annual crops and forest canopies, there is also a linear relationship between biomass production and canopy light interception for horticultural crops, even though the horticultural yield is measured as fruit weight rather than biomass (Figure 12.30. Thus in all managed cropping systems, a universal relationship between canopy light interception and yield or biomass production and the LAI, whether based on continuous or discontinuous canopies, underpins this relationship through determining the extent of light interception by the canopy.Although the upper limit of orchard dry matter production is set by light interception as outlined above, crop composition is determined by sunlight distribution within the canopy, and shade can reduce fruit quality. This is a general phenomenon among perennial fruit crops and has been reported for apple, citrus, peach, cherry, kiwifruit and raspberry. In apple, for example, fruit from more shaded regions are smaller, and have less red colour and lower soluble solids. There are also effects on apple quality after storage, with shaded fruit of Cox’s Orange Pippin tending to suffer more from shrivel and core flush and less from bitter pit than fruit from well-exposed regions of the tree. Fruit of satsuma mandarins grown in shaded regions of the trees are smaller, with a higher acid content and lower sugar content than fruit grown in well-exposed parts of the canopy.
With both peaches and cherries, shading has been found to reduce fruit colour, soluble solids and fruit size and delay abscission and maturity. Kiwifruit from shaded regions of the canopy, or individual fruit which has been artificially shaded during the season, show reduced fruit fresh weight, soluble solids, firmness and chlorophyll concentration in the mesocarp compared to well-exposed fruit. With grapevines, too, shaded fruit have generally lower sugar, total phenol and anthocyanin but higher pH, malate, potassium and titratable acidity than fruit grown in well-exposed positions in the canopy. These differences in grape quality have carried through to the wine quality made from the grapes. A consequence of decreased sugar levels in shaded grapes is a delay in harvesting. This may put crops at risk in climatically marginal regions.
Generalisations on sunlight and fruit quality have been derived from shading experiments on whole trees or parts of trees, or from correlation studies of fruit quality and irradiance in different parts of the canopy. Consequently both fruit and foliage have been subjected to reduced light, but some features in grapes and apples, such as anthocyanin development, vary according to illumination of the fruit itself. With grapes, cluster shading reduces fruit anthocyanin and total soluble phenolics, while leaf shading results in smaller berries with lower glucose and fructose content.
Shade within orchard trees can arise from self-shading or from adjacent trees or shelterbelts. Light levels in midsummer within well-spaced, traditionally pruned large peach trees can drop to 4–15% of incoming radiation. Sour cherry trees tend to be more dense, and light levels as low as 2–4% have been reported. Vigorous grape canopies can be very dense, with irradiance within the canopy reduced to less than 1% of ambient. With such dense canopies, between-row shading will be unacceptable if the ratio between canopy height and clear alleyway spacing exceeds 1:1 (Smart 1989). With the much more open canopies of apples, such ratios may be less useful, as acceptable light distribution within the trees can be obtained over a wide range of ratios of canopy height to clear alleyway spacing. With apple canopies, a ratio between actual light interception and \(F_\mathrm{max}\) may be more useful. If this ratio exceeds 0.7 then self-shading is probably excessive.
Overall effects of shade on fruit quality are very clear, but processes responsible are not so clear. Shade reduces PAR and, therefore, local photosynthetic activity; but canopy shade also reduces temperature (Greer and Weedon 2012) and changes wavelength distribution of transmitted light. Leaves absorb strongly in the visible part of the spectrum (400–700 nm), but have a high transmittance and reflectance to wavelengths in the near infrared (see Figure 12.1 at start of this chapter). This difference in light quality between incident and transmitted sunlight is usually expressed as a red/far-red ratio, that is, a ratio between irradiance around 660 nm compared with 730 nm wavebands. These wavebands correspond to peaks of absorption by phytochrome (Chapter 8), and are strongly implicated in developmental responses to canopy shade. Correlated changes in irradiance and the red/far-red ratio of canopy light make it difficult to separate the effects of changing irradiance from the effects of a change in the red/ far-red ratio, but it is likely that changes in berry composition are due to combined effects of irradiance and red/far-red ratio.
Due to the perennial nature of fruit trees, environmental conditions in one season can have carry-over effects on growth and development during the subsequent season. In seasonally deciduous trees and vines, flowers that emerge each spring were actually initiated during the previous growing season, and conditions prevailing at that time will have influenced the extent and intensity of flower bud differentiation. In temperate horticulture, flower initiation is not a continuous process but may be restricted to several weeks during mid to late summer, followed by several months of continuing development before passing into dormancy over winter. Floral development is then completed during the following spring ahead of flower emergence. In addition to changes in fruit quality of current crops, canopy shade during summer will also reduce flower bud initiation and differentiation.
Flower bud reduction due to shading seems to be a general phenomenon in perennial fruit crops and has been reported for apple, pear, apricot, peach, coffee, cacao and grape. With grape, the number and size of cluster primordia generally increases with increasing light. In this case, plants are responding to light quantity rather than quality (expressed as changes in the red/far-red ratio). Cultivars differ in their response to shade. Sultana (syn. Thompson Seedless) requires approximately 30% full sun for cluster initiation compared to 10% for Rhine Riesling. Timing of shading is also important for grapes. Shading in the period during bud development of grape in late spring has the greatest negative effect on flower initiation.
In citrus, shading may not of itself reduce flowering but can induce heavy fruit abscission. Similarly, shading whole apple trees 14–28 d after full bloom can result in very heavy fruit loss. Two days shading with shade cloth transmitting 8% light was sufficient to reduce fruit set by 80% (Byers et al. 1991). So effective is this treatment that deep shade could work as a non-chemical fruit thinner! In sour cherry, fruit set is adversely affected by light levels within the canopy below 20%, and flower bud initiation requires an irradiance of 15–20% full sun. Shading of whole vines or shoots of kiwifruit during summer can reduce flowering the following spring. Again, as with grapes, changes to the red/far-red ratio did not alter the flowering response of kiwifruit vines to total irradiance. Yields of kiwifruit vines next to shelterbelts are significantly lower than those from vines in the centre of fruit blocks, due presumably to a reduction in flowering as a result of shade from the shelterbelt. This can depress yield by two thirds.
While shading generally reduces fruit quality, excess solar radiation can lead to serious downgrading of fruit quality. Apples, grapes and kiwifruit suffer from sunburn, particularly where the fruit has been growing in the shade and then is thrust into full sunlight by movement of branches due to weight of fruit or to summer pruning or defoliation removing foliage that previously shaded the fruit. This tends to be a much larger problem in regions of high solar radiation receipt than cloudy areas.
Perennial fruit orchards do offer great potential for modification of light and temperature environments within canopies by pruning, tree training, tree size, row spacing and row orientation. Because of the great variety of ways of arranging the foliage in space, a number of models have been written to examine the relative importance of some of these factors in influencing not only light interception (Table 12.1) but also sunlight distribution within the canopy (Palmer 1989; Wagenmakers 1991), as this can have such a profound effect upon the quality of the fruit as already outlined.
In addition, field experiments to examine these effects are very expensive and time consuming to set up and run, so models offer a very economical way of examining light interception and distribution within innumerable canopy forms. As there is often a large dollar premium for quality, the economic success of any production system is largely determined by the yield of high-quality fruit, rather than the total yield. That does not mean, however, that high total yield and high-quality yield are mutually exclusive. With grapevines, Smart (1989) has consistently argued that high yields of high-quality grapes are achievable via correct canopy management.
Highly productive orchards and vineyards, therefore, have a balance of vegetative and reproductive growth, with fruiting zones maintained in a high light environment. Examples of how this has been achieved will now be presented for apples, peaches and grapes. In each case only one system is described, although there are many other successfully managed systems, particularly in vineyards.
In New Zealand, Tustin et al. (1990) have sought to combine requirements for precocity, high yields of high-quality fruit and good light penetration into the canopy in their slender pyramid tree form. This has been developed with trees on semi-vigorous rootstocks at a tree density of about 700 trees per hectare. The form is basically a central leader tree, that is, one central vertical trunk, on which are borne the fruiting branches. Early tree management is aimed at developing a permanent, strong, spreading basal tier of four to five branches emanating from the central leader at wide angles for strength and to encourage early fruiting. Pruning is minimal in the early years, with unwanted shoots being removed during the growing season when they are still small. The upper part of the tree is developed as an open, well-spaced arrangement of whorls of shorter branches arising directly from the central leader. These upper branches are removed when they become too large or pendulant and are replaced by natural regrowth. Each tree is maintained in an overall pyramidal form to encourage light penetration into all parts of the canopy. Yields of Royal Gala apples, for example, have been over 50 t ha–1 in the third year rising to over 100 t ha–1 by year six.
Trees on a Tatura trellis (Figure 12.27) are trained into two planar canopies inclined at an angle of 60º from the horizontal, and held by a trellis system of posts and wires. The system is designed to encourage precocity by using 2,000 trees per hectare and rapidly filling the trellis structure with fruiting branches. Within each arm, the canopy itself is shallow to encourage good light penetration. Summer pruning of unwanted, upright vegetative growth is practised to maintain a high, uniform light interception environment along each arm. Vegetative growth has also been successfully controlled in the dry environment of Tatura by regulated deficit irrigation.
The Tatura trellis is also designed for mechanical picking. A rigid and shallow canopy ensures that fruit can fall onto the catching frame with minimal chance of damage. As peaches bear fruit on one-year-old wood, yields of Golden Queen on Tatura trellis in year two have reached 28 t ha–1 rising to a maximum of 86 t ha–1 by year four (van den Ende et al. 1987). Other stonefruit and pomefruit (pipfruit) have also been grown successfully with this management system.
Traditional pruning of grapevines was aimed at producing a limited number of moderate-sized clusters of berries to facilitate hand picking. Consequently, pruning removed large amounts of young wood so that growth of vigorous shoots was stimulated. With the advent of mechanical picking for wine grapes, however, this constraint has been removed as the harvesters are capable of efficiently removing the berries from large and small grape bunches. This has consequently brought about new grapevine pruning and training techniques, particularly in regions with long growing seasons, irrigation and high vigour such as Australia, Chile and California.
Minimal pruning of cordon-trained vines (MPCT) is one such example from Australia. Each vine is initially developed conventionally with two or four permanent horizontal arms or cordons. From year three, vines are minimally pruned — low hanging canes are removed. Consequently MPCT vines carry far more buds over from one year to the next, but competition between these growing points results in shorter shoots, with shorter internodes, and higher yields made up of a large number of small clusters of berries well exposed to the light. The resulting wine quality is high and comparable with that from conventionally pruned vines.
The solar UV spectrum (Figure 12.31) extends from 100 to 400 nm, and has been divided into three bands: UV-A, 315 – 400 nm; UV-B, 280 – 315 nm; and UV-C, 100 – 280 nm. These divisions are somewhat arbitrary (the UV-A/UV-B boundary ranging from 315 to 320 nm and the UV-B/UV-C boundary from 280 to 290 nm) and relate to effects that each band has on biological systems.
The shortest UV wavelengths reaching ground level are in the UV-B range (280–320 nm). This wavelength range represents up to 1.5% of extraterrestrial irradiance, and it is attenuated to 0.5% or less of total irradiance reaching the earth’s surface (Figure 12.31). While UV-B is only minor in terms of total solar irradiance at ground level, the high energy of UV photons make UV-B a photo-chemically active and biologically significant form of radiation. Proteins, DNA and RNA, absorb UV-B radiation strongly (Figure 12.31) and are thus prone to damage. As production and destruction of stratospheric ozone is largely dependent on absorption of solar UV, the ozone ‘layer’ essentially shields the earth’s surface from most UV radiation. However, even small increases in UV-B irradiation arising from depletion in stratospheric ozone could have significant effects on biological systems.
Figure 12.31 Upper portion, Spectral irradiance of terrestrial and extraterrestrial solar radiation together with that generated by a typical UV sunlamp. The shift to lower UV wavelengths of terrestrial radiation due to ozone depletion generalised UV action spectrum for plant damage is shown. Lower portion, Relative absorption of UV radiation by nucleic acids, proteins and flavoproteins. (Original diagram M.M. Caldwell)
UV-B irradiance rose markedly during the 1960s, due to ozone depletion. In addition, South polar stratospheric ozone suffers enhanced depletion each southern hemisphere spring, and the south polar 'ozone hole' region reached an area of about 24 million square kilometres on 7 October 1994.
A question remains about the causes of the ozone hole. Notwithstanding a consensus on stratospheric chemistry and UV-B among atmospheric scientists, could the ozone hole be a ‘natural’ phenomenon?
Figure 12.32 Reciprocal variation in atmospheric ozone over the South Pole (open symbols), and flavonoid content of the moss Bryum argenteum (solid symbols). Ozone concentration is indicated as Dobson units which represent the physical thickness of the ozone layer at a pressure of one atmosphere (e.g. 300 Dobson units = 3 mm). (Original data R.K. Markham)
Ground-based measurements of ozone over the South Pole made between 1964 and 1986 imply that ozone depletion over the Antarctic is not necessarily a consequence of recent human activity and may have been influenced by ‘natural’ processes. This view was supported by measurements of flavonoid levels in samples of the moss Bryum argenteum (Figure 12.32). Flavonoids are synthesised in Bryum as a UV-B-screening pigment and synthesis is sensitive to small changes in UV-B irradiation. Samples of Bryum collected from the Ross Sea area between 1957 and 1989 showed that flavonoid content rose markedly during the mid-1960s. This was correlated with a reduction in ozone levels at the time, but ahead of any serious accumulation of CFCs (chlorofluorocarbon compounds) that are considered to affect the atmospheric concentrations of ozone. Key agents in this mid-1960s ozone depletion are believed to include altered sunspot activity, emissions from volcanic eruptions and atmospheric tests of nuclear weapons in the early 1960s.
Investigations of UV effects on plant biology largely depend on the use of UV-B emitting lamps in both laboratory and field. Interpretation of results from such systems is, however, restricted by an inability to mimic the solar spectrum precisely (Figure 12.31). As a consequence, comparisons between species with respect to UV-B sensitivity, and analyses of processes responsible for variation in sensitivity, must be treated circumspectly. Despite these methodological limitations, overall outcomes are unambiguous: UV-B radiation impacts on many aspects of plant biology, as reviewed by Tevini (1994) and Bornman and Teramura (1993).
Sensitivity to UV-B irradiation includes any morphological, physiological or biochemical change induced by UV-B. Significant effects have been reported for about half of the 300 or so crop species and cultivars studied so far, with some species more susceptible than others (Table 12.2). Sensitivity is noticeable in plant growth and development. Reduction in leaf area, stem growth (stunting) and total plant biomass are commonplace. Additional symptoms include bronzing and glazing of leaf surfaces.
Growth reductions depend not only on the level of UV-B exposure but on the associated photon irradiance. Partitioning of growth to different organs, for example altered internode length, or leaf production, is also sensitive to UV-B irradiation. Such morphological changes may not be significant to plants in isolation but may influence competitive interactions, and especially their competition for sunlight. Sensitivity to UV-B irradiation also varies with life cycle. Seedlings are especially vulnerable, as are mature plants under-going their transition from vegetative growth to reproductive development.
Several sets of processes at both cellular and molecular levels which are critical to plant growth and reproductive development appear to be affected by absorption of UV-B radiation. Especially prominent are photosynthesis, signal transduction and nucleic acid metabolism.
In general, UV-B radiation causes a net inhibition of photosynthesis in a wide range of plants. From laboratory studies, this inhibition appears to arise from disruptions at a number of points in the photosynthetic cycle, including disruption of PSII reaction centres (Strid et al. 1990), a decrease in Rubisco activity and damage to photosynthetic pigments (chlorophylls and carotenoids). Stomatal function, and thus leaf gas exchange, is also commonly impaired.
Alterations in plant growth induced by UV-B radiation have been partly attributed to changes in nucleic acid structure and function (Tevini 1994). DNA absorbs strongly in the UV region and is thus especially prone to damage by UV-B radiation (Figure 12.31). The most common lesions are breaks in DNA chains, with a resultant production of thymine dimers. They in turn interfere with accurate base pairing, leading to a disruption of transcription and replication of DNA (Stapleton 1992). These disruptions amount to a mutagenic action for UV radiation in many organisms. Proteins and RNA can also absorb UV-B and are inactivated as a result, but this loss is of secondary importance due to their relative abundance compared with DNA (Stapleton 1992).
Activation and photo-deactivation of important signal molecules, such as hormones and photoreceptors, may also compound effects of UV-B irradiation on plant growth and development. For example, cell extension in many plants is influenced by indole acetic acid (IAA) which absorbs in the UV-B region and is photo-oxidised to 3-methyleneoxindole, an inhibitor of hypocotyl growth when exogenously applied. In contrast, irradiation with UV-B can induce enzyme activity in the shikimic acid pathway, which regulates the synthesis of a broad array of plant compounds, ranging from flavonoids to lignin, all of which are important to plant function, including tolerance to UV-B radiation.
Flowering, pollination and seed development are variously affected by UV-B irradiation. Flowering appears to be disrupted by UV-B radiation by decreases in flower number and alteration in timing reported in the few species so far studied. Such effects may have important consequences for natural plant populations, which rely on the synchrony of flowering with the presence of appropriate insect pollinators.
Although pollen appears resilient to UV-B radiation damage, owing to high levels of flavonoids in the anthers and pollen wall, germinating pollen tubes are highly sensitive to UV radiation and suffer from large decreases in growth rate. Such disruption can lead to lowered fertilisation success and decreases in seed yield.
Genetic variation in sensitivity to UV-B radiation has implications for plant competition and thus plant ecosystem dynamics and community structure, in both natural and managed ecosystems. Competition between agricultural crops and associated weeds may be altered under enhanced UV-B irradiation. In the few studies considering the impact of UV-B irradiation on natural plant communities, most notable is the work of Caldwell et al. (1982). They found arctic plant species were much more sensitive to UV-B, both in terms of growth and reproduction, than alpine species. Arctic species grow under a naturally low UV-B environment, whereas alpine species grow under a naturally high UV-B environment by virtue of higher altitude. Arctic plant communities could thus be altered to a greater extent than alpine communities by any substantial rise in natural UV-B.
Many organisms have evolved mechanisms to undo the molecular damage caused by UV radiation. Possibly the most adaptive are terrestrial plants that rely on full sunlight for photosynthesis. Protective mechanisms can be classified into two main classes: (1) those whereby UV damage is repaired or its effects negated or minimised, and (2) those whereby the amount of UV radiation actually reaching sensitive areas is reduced. While protective in nature, all of these mechanisms impose an energy cost on plants so adapted.
For the first class of protective mechanism, organisms have developed three important repair processes for UV-induced DNA damage (Stapleton 1992): photoreactivation, excision and repair of DNA, and post-replication repair. Photoreactivation is the light-induced enzymatic process which cleaves pyrimidine dimers formed by UV radiation — thus restoring proper base pairing. Excision and repair of DNA involves the excision of UV photoproducts from DNA molecules (this mechanism requires no light energy and uses undamaged DNA templates as a guide). Post-replication repair occurs where DNA lesions are bypassed during DNA replication and information from the sister duplex is later used to fill gaps.
As mentioned above, plant growth and development is slowed by exposure to enhanced UV-B radiation. Such delay also minimises adverse effects from damage that has occurred. Growth delay and slower cell division provides additional time for DNA repair mechanisms to operate ahead of any recommencement in DNA replication.
Screening sensitive tissues from UV-B radiation is a secondary option available to plants either to avoid or at least minimise damage. Tissue screening may be achieved through structural modification of organs or by screening molecules which absorb UV radiation. Such features may be either static, as with leaf orientation or phototaxis, or dynamic, as in synthesis of screening molecules which absorb UV radiation in a highly selective way. Screening molecules commonly appear after exposure to UV radiation as secondary metabolites such as flavonoids. Substantial amounts of such pigments accumulate especially in the upper epidermis of leaves. Along with cuticular waxes and other cellular components, these substances attenuate incident UV radiation, and energy transmitted to underlying tissue is decreased by up to two orders of magnitude (Figure 12.33). Typically less than 1% of incident UV-B radiation reaches the lower epidermis. None is transmitted through an entire leaf. Flavonoid pigments are synthesised by leaves on many plants in direct response to UV radiation.
Field-grown plants are subject to variable environmental conditions during their life cycle. In their extremes, such factors can lead to stresses such as water stress, mineral stress, temperature stress, disease and stresses due to anthropogenic pollution (ozone, acid rain). The interaction of UV-B irradiation stress with other environmental factors has been examined in only a few cases, essentially because of the difficulty of simulating elevated UV-B irradiation in the field. Of the environmental interactions studied, water stress appears to influence plant responses induced by UV-B irradiation. In soybean, for example, a decrease in yield in sensitive cultivars under elevated UV-B irradiation was most apparent under well-watered conditions. Water deficit in combination with elevated UV-B irradiation did not reduce yield to a greater extent than water deficit alone. Water stress may, therefore, mask UV-B responses of plants.
Elevated CO2 levels may also modify the response of sensitive plants to UV-B irradiation. In rice and wheat (but not in soybean) an increase in seed yield and biomass under elevated CO2 was reversed under moderate levels of supplementary UV-B irradiation.
Vascular plants have evolved over millennia with features that now enable utilisation of light in climates that differ by orders of magnitude in photon irradiance. Their adaptation to sun and shade is a marvel of nature’s biological engineering, with a wide range of adaptive features at all levels of organisation from chloroplast to community. Moreover, the nature of selection pressures imposed by sunlight has also changed. The blistering UV radiation of a prebiotic world has been attenuated thanks to oxygenic photosynthesis, and vascular plants still carry adaptive features that may again find strong expression if global change does result in a substantially greater UV-B irradiance.
The central significance of sunlight as an energy source for natural and managed ecosystems is unarguable, and quantitative relationships between forest and horticultural productivity and sunlight interception provide just examples that underscores human reliance on intrinsic properties of photo-energetics. More subtle, and especially costly in terms of gaining necessary research experience, is plant response to light quality.
Optimising interception of sunlight by managed communities of plants in horticulture calls for application of solid geometry, and some highly sophisticated canopy systems have been developed. However, a knowledge of growth and developmental response to changes in spectral composition of sunlight transmitted by plant canopies provides crucial insight into reproductive physiology. Concord grapevines were cited as an example of a species with features that make them especially amenable to canopy manipulation. Having evolved in North America as forest vines, vegetative extension rather than reproductive development would have conferred a selective advantage for reaching exposed crowns in forested habitats. Accordingly, the far-red-enriched sunlight transmitted by their own canopy in a managed vineyard encourages shoot extension, rather than differentiation of flower buds and subsequent cropping. That predisposition to vegetative vigour was successfully countered by shrewd management of vine canopies via pruning, trellising and shoot positioning.
Overall, sunlight is pervasive by driving genotype × environment interactions for both evolution of adaptive features and day to day physiology of vascular plants. Our growing appreciation of photobiology in managed communities, and of nature’s adaptations in natural communities, has already paid huge dividends in understanding plant function. What is even more compelling is that such knowledge will shape our future options for plant utilisation.
Anderson MC (1970) Radiation, climate, crop architecture and photosynthesis. In I Setlik, ed, Prediction and Measurement of Photosynthetic Productivity. Pudoc, Wageningen, pp 71–78
Bornman JF, Teramura AH (1993) Effects of UV-B radiation on terrestrial plants. In AR Young, LO Björn, W. Nultsch, eds, Environmental UV Photobiology. Plenum Press, New York, pp 427–471
Byers RE, Carbaugh DH, Presley CN, Wolf TK (1991) The influence of low light on apple fruit abscission. J Hort 66:7-17
Caldwell MM, Robberecht R, Billings WD (1982) Differential photosynthetic inhibition by ultraviolet radiation in species from the artic-alpine life zone. Artic Alpine Res 14: 195-202
Chazdon RL, Pearcy RW (1986) Photosynthetic response to light variation in rainforest species. I. Induction under constant and fluctuating light conditions. Oecologia 69: 517-523
Chow WS (1994) Photoprotection and photoinhibitory damage. In J. Barber, ed, Advances in Molecular and Cell Biology: Molecular Processes of Photosynthesis, Vol. 10, JAI Press Inc.: Greenwich, Connecticut, pp 151–196
Jackson JE (1980) Light interception and utilization by orchard systems. Hort Rev 2: 208–267
Greer DH (1996) Photosynthetic development in relation to leaf expansion in kiwifruit (Actinidia deliciosa) vines during growth in a controlled environment. Aust J Plant Physiol 23: 541 – 549
Greer DH, Weedon MM (2012) Interactions between light and growing season temperatures on growth and development and gas exchange of Semillon (Vitis vinifera L.) vines grown in an irrigated vineyard. Plant Physiol Biochem 54: 59 – 69
Hay RKM, Porter JR (2006) The Physiology of Crop Yield. 2nd Edition, Blackwell Publishing: Oxford, UK.
Khurana, SC, McLaren JS (1982) The influence of leaf area, light interception and season on potato growth and yield. Potato Research 25, 329-342.
Kiniry JR, Jones CA, O’Toole JC, Blanchet R, Cabelguenne M, Spanel DA (1989) Radiation-use efficiency in biomass accumulation prior to grain-filling for grain-crop species. Field Crops Res 20: 51–64
Kriedemann PE, Torokfalvy E, Smart RE (1973) Natural occurrence and photosynthetic utilisation of sunflecks in grapevines. Photosyn 7: 18-27
Lang ARC, Xiang YQ, Norman JM (1985). Crop structure and the penetration of direct sunlight. Agric Forest Meteorol 35: 83–101
Long SP, Humphries S, Falkowski PG (1994) Photoinhibition of photosynthesis in nature. Annu Rev Plant Physiol 45: 633-662
Lumsden P (ed.) (1997) Plants and UV-B. Responses to Environmental Change. Soc Exp Biol Seminar Series 64, Cambridge University Press
Monteith JL (1977) Climate and the efficiency of crop production in Britain. Phil Trans Royal Soc Series B 281: 277-294
Monteith JL Unsworth MH (1990) Principles of environmental physics. Edward Arnold: London
Palmer JW (1989) The effects of row orientation, tree height, time of year and latitude on light interception and distribution in model apple hedgerow canopies. J Hort Sci 64: 137-145
Pfitsch WA, Pearcy RW (1989) Daily carbon gain by Adenocaulon bicolor (Asteraceae), a redwood forest understory herb, in relation to its light environment. Oecologia 80:465-470
Shaulis N, Jordan TD, Tomkins JP (1966). Cultural Practices for New York Vineyards, Cornell Extension Bulletin 805, New York State College of Agriculture: Geneva, New York.
Smart RE (1989) Solar radiation interception as a guide to the design of horticultural plantings. II. Twenty years of experience with grapevines. Acta Hort 240: 87-94
Stapleton AE (1992) Ultraviolet radiation and plants: burning questions. Plant Cell 4: 1353-1358
Strid A, Chow WS, Anderson JM (1990) Effects of supplementary ultraviolet-B radiation on photosynthesis in Pisum sativum. Biochim Biophys Acta 1020: 260-268
Tevini M (1994). UV-B effects on terrestrial plants and aquatic organisms. Progress Bot 55: 174–190
Thompson WA, Kriedemann PE, Craig IE (1992a) Photosynthetic response to light and nutrients in sun-tolerant and shade-tolerant rainforest trees. I. Growth, leaf anatomy and nutrient content. Aust J Plant Physiol 19: 1-18
Thompson WA, Kriedemann PE, Craig IE (1992b) Photosynthetic response to light and nutrients in sun-tolerant and shade-tolerant rainforest trees. II. Leaf gas exchange and component processes of photosynthesis. Aust J Plant Physiol 19: 19-42
Trenbath BR, Angus JF (1975) Leaf inclination and crop production. Field Crop Abstracts 28: 231–244
Tustin DS, Corelli-Grappadelli L, Ravaglia G (1992) effect of previous-season and current light environments on early-season spur development and assimilate translocation in Golden Delicious apple. J Hort 67:351-360
Van den Ende B, Chalmers DJ, Jerie PH (1987) Latest developments in training and management of fruit crops on Tatura trellis. Hort Sci 22: 561-568
Wagenmakers PS (1991) Planting systems for fruit trees in temperate climates. Crit Rev Plant Sci 10: 369–385
Watling JR, Ball MC, Woodrow IE (1977a) The utilization of sunflecks for growth in four Australian rainforest species. Funct Ecol 11: 231-239
Watling JR, Robinson SA, Woodrow IE, Osmond CB (1977b) Responses of rainforest understorey plants to excess light during sunflecks. Aust J Plant Physiol 24: 17-25.
Wünsche JN, Lakso, AN, Robinson TL, Lenz F, Denning SS (1996) The bases of productivity in apple production systems: The role of light interception by different shoot types. J Amer Soc Hort Sci 121: 886-893