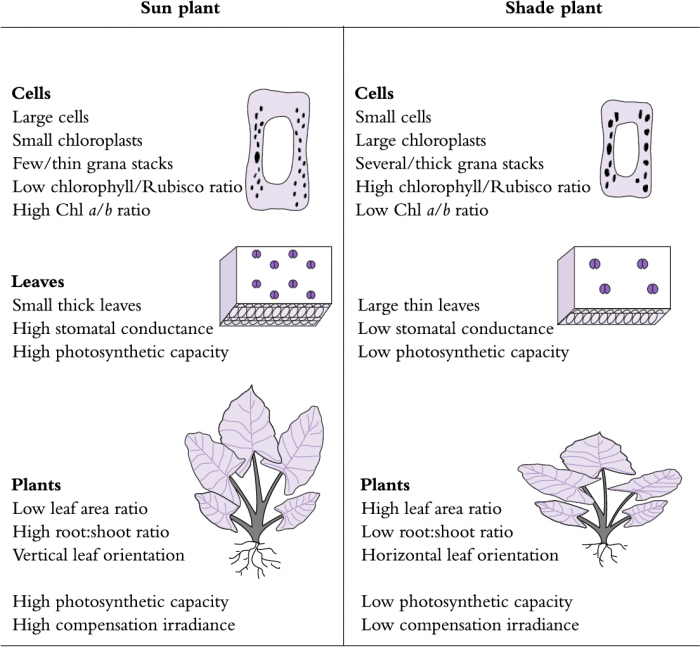
Figure 12.2 A comparison of photosynthetic structure and function in sun and shade plants. Major characteristics are described for cells, leaves and whole plants. (Original drawing courtesy S.A. Robinson)
In low light, plants need to absorb maximum light for photosynthesis if they are to survive. In high light the problem is reversed. Plants need to maximise their capacity for utilising their abundant light energy. At the same time, the plants have to deal with excess sunlight when their photosynthetic capacity is exceeded. As a consequence of such unrelenting selection pressures, plants have evolved a variety of features that optimise light interception, absorption and processing, according to the light environment in which they had evolved and adapted (Figure 12.2). Adaptation implies a genetically determined capability to adjust attributes, i.e., acclimate to either sun or shade. Such acclimation calls for adjustment in one or more attributes concerned with interception and utilisation of sunlight. Common features of either sun or shade plants are outlined below, and the advantage to plants growing in different light environments is discussed. Field applications are illustrated with examples of sun/shade acclimation and sunfleck utilisation in rainforest plants.
Initial steps of photosynthesis involve interception and absorption of photons by photosynthetic organs; subsequent steps are involved with utilisation or dissipation of the absorbed quantum energy. Interception of light varies according to size, angle, orientation and surface features of the photosynthetic organ(s) and is also influenced by the arrangement of photosynthetic tissue within those organs (Figure 12.2).
Many plants can change their leaf angles and orientation in response to a change in light. For some, to increase interception while for others, to avoid high light. A good example of optimising light interception through leaf movement is given by Oxalis oregana, an understorey herb of redwood forests in western USA (Figure 12.4). This plant is able to track sunlight on dull days, but can change leaf angle from horizontal to vertical in only 6 min, if exposed to full sunlight. In this way, leaves can maintain maximum photosynthetic rates under a variety of light conditions but can avoid photoinhibition of photosynthesis by leaf folding. Omalanthus novo-guinensis, an Australian rainforest plant, can also change leaf angles within about 20 min in response to full sunlight (Watling et al. 1997b).
Another way of reducing light capture is a change in leaf-surface properties. Many plants in high light environments have a high reflectance of their leaves from a coat of hairs or wax or even salt crystals. Cotyledon orbiculata, a crassulacean acid metabolism (CAM) plant from southern Africa, produces a wax coating on the leaves. Plants grown at high light produce copious quantities of white wax which reflects 60% of incident light whereas plants grown in low light produce very little wax and leaf reflectance drops to 9% (Figure 12.5). Young eucalypt leaves also produce wax, while leaves of Celmisia longifolia, the snow daisy of the Australian Alps, are covered in a thick layer of silvery fibres. In these instances, plants are avoiding high light by creating their own shade, but does leaf anatomy adjust to environments where light is limiting?
Epidermal cells in some rainforest shade-adapted species are shaped to enhance light capture by acting as a lens. The optical properties of such cells focus incident sunlight into the layer of photosynthetic tissue just below the epidermis, reducing light lost due to reflectance and transmittance.
Light interception can also be regulated at a tissue and organelle level. Photosynthetic tissue can be concentrated equally on both sides of a leaf (isobilateral) to maximise light absorption from either side, or preferentially on one side (dorsiventral) as is common in species where leaves are predominantly horizontal.
Chloroplast density and location within leaves is also sensitive to the light climate, and energy capture varies accordingly. Alignment along vertical cell walls will reduce overall absorption of incident light, and in Oxalis leaves absorbance can be reduced 20% when chloroplasts align less to the horizontal and more to the vertical walls of mesophyll cells.
Once sunlight has been intercepted by an assimilatory organ, photon absorption then depends on the extent and nature of light-absorbing pigments in the photosynthetic tissues. In terrestrial plants, the major light-absorbing pigments are chlorophylls a and b plus a range of carotenoids which can act as accessory pigments. Compared with high-light plants, plants grown in low light tend to allocate relatively more resources to their light-harvesting pigments and the associated proteins than to the enzyme Rubisco and other soluble proteins involved in CO2 fixation. This shift in allocation of nitrogen-based resources can be accompanied by marked changes in leaf anatomy, especially depth of mesophyll tissue (see Case study 12.1) and reflects a need for increased efficiency of light absorption when sunlight is limited.
There are also differences in chloroplast structure between plants grown in low light and high light. Shade chloroplasts tend to be larger than those found in sun plants. They also contain more thylakoid membranes which show higher levels of randomly arranged granal stacking into appressed regions, as shown by the extreme development of grana in Figure 12.6. The higher proportion of appressed to non-appressed membranes found in shade chloroplasts is the result of increased photosynthetic system II (PSII) and antenna (LHCII) content. LHCII is thought to be involved in thylakoid appression and formation of granal stacks. Plants grown in low light also tend to have lower Chl a/b ratios. Chlorophylls a and b are both associated with the light-harvesting antennae, while only Chl a is found in the reaction centres. A lower a/b ratio, therefore, reflects an increase in LHCII complexes relative to reaction centres (see Chapter 1, Section 1.2).
In addition to differences in leaf anatomy and chloroplast fine structure, energy derived from absorbed sunlight is processed in ways that differ subtly between shade-grown and sun-grown plants. In high light, there is a requirement for greater capacity in both the light and CO2 fixation reactions of photosynthesis. Photosynthesis–light response curves for shade and sun plants (Figure 12.7) illustrate such differences. The initial slope of each light response curve represents the quantum or photon efficiency of photosynthesis. This is the same for sun and shade plants. The reason it does not change is that the efficiency of the light reactions is the same irrespective of how much light has been received during growth (i.e. eight photons are required for the evolution of one molecule of O2 and fixation of one molecule of CO2 in all plants).
Figure 12.7 Photosynthesis-light response curve for typical shade and sun plants, showing relationships between photosynthetic rate and absorbed light (expressed as a photon irradiance). Dashed lines are extrapolations of initial linear slopes where photosynthesis is light limited, and represent quantum yield (moles of O2 evolved per mole quanta absorbed). (Original data courtesy S.A. Robinson)
However, sun plants tend to have a greater capacity for photosynthetic electron transport (greater abundance of transport components such as Cyt b559, Cyt b563, Cyt f and plastoquinone). They also have a greater capacity for ATP synthesis per unit of chlorophyll compared with shade plants. Taken together, these capacities of sun plants allow more sunlight to be processed into ATP and NADPH for use in CO2 assimilation and other synthetic events. Such capacity is also matched by a greater investment in enzymes of the photosynthetic carbon reduction (PCR) cycle, resulting in a higher light-saturation point and a higher maximum rate of photosynthesis (Pmax) for sun plants (Figure 12.7). As a further distinction, sun leaves tend to be thicker and have more cell layers. They also have higher stomatal conductances to facilitate rapid uptake of CO2.
A higher photosynthetic capacity in sun plants does, however, incur some costs. The sun leaves tend to have higher respiration rates which increases the light-compensation point relative to shade plants (Figure 12.7). Higher respiration rates probably result from (1) increased carbohydrate processing in high light, (2) increased costs of constructing sun leaves and (3) a higher cost of maintaining sun leaves. Further details on maintenance costs are given in Chapter 5, Section 6.5.
Greater transpiration is a further cost of the higher photosynthetic capacity as a result of higher stomatal conductance. Sun plants often respond to the greater transpiration by increasing their root : shoot ratios. Under conditions where water is limiting, however, stomatal conductance may be reduced, sacrificing photosynthesis in favour of slower transpiration.
In the light-response curves for photosynthesis (Figure 12.7 above), photosynthesis is regarded as light limited in the initial linear region of the curve. However, at higher photon irradiances once the light-saturation point (that is, the maximum rate of photosynthesis) has been reached, further increases in light will exceed the energy-utilising capacity of that photosynthesising leaf. Refer to the dashed lines in Figure 12.7 which represent a continuation of the initial rate of photosynthesis (quantum yield of photosynthesis) and demonstrates the actual light absorption. The extent to which this absorbed light is not ‘gainfully employed’ for photosynthesis is set by Pmax (the light-saturated rate of photosynthesis in normal air). At low light (< 100 µmol quanta m–2 s–1), both sun and shade leaves use more than 80% of the absorbed light for photosynthesis. However, once Pmax has been reached, all additional absorbed light is in excess of that which can be used in photosynthesis.
Since shade plants have a lower Pmax than sun plants, they experience more excess light at a given photon irradiance above saturation. Additional stresses such as drought, nutrient limitation or temperature extremes can also lead to a reduction in Pmax and thus increase the probability of plants being exposed to excess light. However, even the most hardy sun plant will reach Pmax at less than full sunlight (incident beam normal to leaf surface). At that level (say, 1000 µmol quanta m–2 s–1), approximately 25% of absorbed energy is used to drive photosynthesis, but at full sunlight (c. 2000 µmol quanta m–2 s–1) as little as 10% is used (Long et al. 1994). Individual leaves on plants growing in full sun commonly experience such excess light intensities. This is potentially damaging, and plants adapted to full sunlight have evolved a number of mechanisms for either avoiding excess light or for dissipating the excess absorbed energy.
Figure 12.8 As photon irradiance is increased, utilisation of energy gives way progressively to dissipation of energy. Photosynthetic events shift from photochemistry, to external and then internal photoprotection and finally to photodamage. Their comparative importance for shade leaves and sun leaves is indicated. Photoprotection is especially well expressed in sun leaves acclimated to additional environmental and biotic stresses. (B. Demmig-Adams and W.W. Adams, Annu Rev Plant Biol 43: 599-626, 1992)
Shade plants have an even greater need to dissipate excess light interception because they absorb more light (more chlorophyll per unit leaf mass), but need less light to saturate photosynthesis. Prolonged exposure of plants to excess light induces photoprotective processes that reduces the photon yield of photosynthesis, but Pmax remains unchanged (curve A). However, further exposures to excess light will result in both photon yield and Pmax being reduced (curve B). The photosynthetic rate is then reduced at all light levels as a consequence of the photoprotection.
Photoprotection is normally sufficient to cope with light absorbed by leaves; the more extreme photodamage only occurs when the capacity for photoprotection is exhausted. Photodamage is manifested as a decline in both photon yield and Pmax, and recovers very slowly (hours to days), whereas photoinhibition and photoprotection recovers much faster (minutes to hours). Severe photodamage results in bleaching of pigments and damage to membranes (photo-oxidation) and may lead to tissue death.
Consider the alternative fates of light energy absorbed by a leaf and their relevance to photoprotection, photoinhibition and photodamage (Figure 12.10). Although blue light has higher energy and causes excitation to a higher excited state, this energy is quickly lost as heat, and chlorophyll molecules drop to a lower excited state. Utilisation of energy from excited chlorophyll molecules results in either assimilatory or non-assimilatory photochemistry, thermal dissipation or release of light of a longer wavelength (fluorescence). The proportion of absorbed energy consumed by these different processes dictates their comparative significance, and in order of importance as protective devices they are:
Most of the NADPH and ATP formed during photosynthetic energy transduction is stored as stable photosynthetic products. Some is consumed in photorespiration and nitrate reduction. Because these non-assimilatory processes also utilise NADPH and ATP, they help reduce the need for photoprotection. The Mehler reaction, in which electrons flow to O2 via photosynthetic system I (PSI) (Chapter 1, Figure 1.10), still supports electron flow and thus might also reduce a need for photoprotection.
However, if photochemical capacity is exceeded by incoming energy, a plant will engage photoprotective mechanisms which increase the amount of energy dissipated as heat. This non-photochemical conversion of light energy is thought to occur in the PSII antennae and involves a group of pigments known as xanthophylls and includes violaxanthin, antheraxanthin and zeaxanthin (Figure 12.11). These are a special group of carotenoid pigments which undergo interconversion in response to excess light. Energy is dissipated in the process. In low light, violaxanthin predominates, but when light is in excess, conversion to zeaxanthin via antheraxanthin occurs. This conversion requires a low pH, ascorbate and NADPH. Such conditions exist in the lumen of chloroplasts in high light. Zeaxanthin, and possibly antheraxanthin, provide photoprotective thermal dissipation of the excess light energy. When light levels are no longer excessive, zeaxanthin slowly converts back to violaxanthin via antheraxanthin (Figure 12.11). Total pool sizes of the xanthophyll pigments increase with increasing exposure to excess light. Sun plants can have three- to four-fold larger pools of violaxanthin, antheraxanthin and zeaxanthin than shade plants and the presence of other stresses can also result in increases in pool size.
Internal differences between sun and shade leaves with respect to energy dissipation are also apparent in the different patterns of attenuation of light through mesophyll tissues, such as in succulent CAM plants such as Cotyledon orbiculata. There, xanthophylls are mostly found in outermost cell layers where the light environment is strongest. If the reflective wax coating is intact, no internal photoprotection is required at the growth irradiance and there is no zeaxanthin formed. However, if the surface wax (external photo-protection) is removed by hand, internal photoprotection is then needed and zeaxanthin appears in the outermost layer (Figure 12.12).
Overall activity of the xanthophyll cycle varies with mesophyll irradiance, while the concentration of particular components also varies according to tissue depth. Changes in concentration of the various components of the xanthophyll cycle are shown here for successive (1 mm) layers in thick leaves of C. orbiculata, with and without their wax coating. In waxed leaves at the end of a dark period (top figure, Figure 12.12) xanthophyll cycle pigments are represented mainly by violaxanthin (V) with a small amount of antheraxanthin (A). After 6 h exposure to high light (middle figure, Figure 12.12) a small amount of violaxanthin is converted to antheraxanthin, but no zeaxanthin (Z) is formed, indicating that the natural wax coating on these leaves is protecting chloroplasts from excess light. Physical removal of surface wax (brushed leaves in bottom figure, Figure 12.12) results in zeaxanthin production within the uppermost layer of leaf tissue but not in deeper tissues or lower surfaces. Restriction of this xanthophyll cycle component to the top section confirms that zeaxanthin accumulation is a response to excess light.A seed germinating in a rainforest understorey starts life in a low light environment. This will not present major problems to an obligate shade species which cannot tolerate strong sunlight; such species have adapted to life in an understorey. However, many rainforest species are better described as either shade tolerant (i.e. able to germinate and persist in low light, but requiring higher light to reach maturity) or shade intolerant (unable to germinate or grow in low light). In successional terms, shade tolerance is a feature associated with climax species and shade intolerance with pioneer species.
Shade-tolerant species can persist as seedlings in the understorey, often for years, while still being able to respond to an increase in light availability when it occurs.
By comparison, shade-intolerant (early-successional) species can only germinate and grow where there is ample sunlight, and consequently they tend to occur in wide gaps and on forest edges. Wide gaps are relatively rare in old-growth rainforests which have been undisturbed by logging or slash and burn agriculture. Shade-intolerant species are unable to maintain a positive carbon balance when growing in low light. The change from a high light to a low light environment requires a change in allocation of plant resources as described in the quantitative growth chapter (Section 6.2). Shade-intolerant plants are unable to make this change and are burdened with the higher costs of construction and maintenance of leaves better suited to strong sunlight
Shade-intolerant species tend to produce numerous small seeds throughout the year which are widely dispersed. Their seeds are also able to remain viable for long periods (years) by going through a period of dormancy. This is often broken by high temperature or strong direct sunlight with a high ratio of red to far-red irradiance (R:FR ratio decreases with sunlight attenuation through canopies). Such environmental cues for germination are all experienced in wide gaps. Following germination, seedlings show rapid growth to maturity, allowing them to become well established in a gap before other slower growing species. These characteristics increase the probability of success for shade-intolerant species in the heterogeneous light environment of a rainforest.
Shade-tolerant species, on the other hand, have evolved a different suite of characteristics. They tend to produce a few large seeds which are generally not well dispersed, with little or no dormancy. However, the seeds have the ability to germinate in low light and persist in the understorey as seedlings for years. A rarity of gaps and a lack of dormancy found in most shade-tolerant species increases the probability of establishing in a low-light understorey environment. In situations like this, the larger seed provides seedlings with a reserve which they can draw upon during early establishment. In rainforests, tree seedlings survival in understorey habitats is positively correlated with seed size, especially in the first few months following germination.
Following establishment in the understorey, seedlings of shade-tolerant species may have to wait a long time before a gap appears overhead. Many species succumb to attack from herbivores or pathogens or may be crushed by large animals (including humans!). Those that do survive must be able to acclimate to the new conditions arising on gap formation; their ability to do this will depend on the nature of the new microclimate and the acclimation potential of each species.
Emergent trees of tropical rainforests have to endure strong sunlight, and leaves comprising the crowns of such trees will have acclimated to full sun. In young-growth forests, canopy emergents are early-successional fast-growing species that are adapted for fast growth in full sun on large disturbances. Such species represent an initial phase in forest dynamics that might last 10–20 years. By contrast, in old-growth forests, early-successional species have long since completed their life cycles, and will have been replaced by later-successional species whose seedlings initially tolerated deep shade on the forest floor, but now endure full sun as canopy emergents. Such remarkable plasticity is an adaptive feature of late-successional species and involves sun/shade acclimation by individual leaves.
The differences in growth rate of early-successional fast-growing species versus later-successional and shade-adapted species is illustrated in Figure 12.13 by two rainforest species that are important in the timber industry: the sun-loving red cedar (Toona australis) and the shade-adapted tulip oak (Argyrodendron sp.).
A detailed study of three rainforest species from North Queensland was conducted by Thompson et al. (1992a, b). The red cedar Toona australis (an early-successional species) and two species of tulip oak Argyrodendron (late-successional species) showed different acclimation potentials when grown under a range of light conditions (Figure 12.14).
When grown under high light, 535 µmol quanta m-2 s-1,which is typical of average canopy radiation in a tropical rainforest, T. australis achieved a higher Pmax and light-saturation point than either of the Argyrodendron species. However, T. australis was more sensitive to nutrient levels, being unable to acclimate to the same degree in low-nutrient compared to high-nutrient regimes. Moreover, fast growth in T. australis was greatly promoted by a positive light x nutrient interaction on leaf expansion and photosynthetic capacity; adaptive features with a clear selective advantage on open sites where soil disturbance liberates nutrients.
Figure 12.14 Photosynthesis versus light response curves for seedlings of a shade-adapted rainforest tree species (Argyrodendron) and a sun-loving tree (Toona australis). Seedlings were grown under factorial combinations of weak, medium or strong light (shown left to right) × either high or low nutrient supply (solid lines with filled symbols, and dashed lines with open symbols respectively). (W.A. Thompson et al., 1992b)
Toona (sun loving) produced a much greater depth of mesophyll tissue under strong light compared to weak light (Figure 12.15b), which accounted for enhanced photosynthetic capacity. Argyrodendron sp. (shade adapted) was much less responsive to photo irradiance during growth, producing consistently thicker leaves regardless of light level, but with lower photosynthetic capacity.
These adaptations result in the more vigorous growth of Toona than Argyrodendron at high light.
Formation of gaps provides an important opportunity for many rainforest plants to escape from the dim understorey environment and reach maturity as canopy trees. Rainforest habitats actually present a continuum of light availability ranging from almost total shade (diffuse light) through intermediate levels of direct and diffuse sunlight to a wide gap where direct sunlight is received for most of a day. Input of direct sunlight beneath a closed canopy can be surprisingly high because of sun patches and more transient sunflecks. Sun patches occur when small and variable openings in the overlying canopy permit direct sunlight to penetrate to the forest floor, resulting in the familiar patchwork of sunlight and shade which can be seen in any understorey on a clear day (Figure 12.16).
Competition for light is intense, and a sunfleck shown passing over this tiny population would be providing a much-needed source of sunlight, namely a few minutes of around 1,500 µmol quanta m-2 s-1 compared with background photon irradiance of around 150 µmol quanta m-2 s-1.
Patches of sunlight move across the forest floor on bright days, and will illuminate any leaves or parts of leaves which lie in their path (Figure 12.17). Daily total photon irradiance on the occasion of the measurement shown in Figure 12.17 was 6.0 mol quanta m-2 d-1). The abrupt increase from a background level of around 50 µmol quanta m-2 s-1 to 1,750 µmol quanta m-2 s-1 energises photosynthesis, but is counteracted by increased leaf temperature, especially during prolonged exposures. Transpiration cooling is a significant component of heat budgets for such large leaves, so that adequate soil moisture is a prerequisite for continuing leaf gas exchange during a sunfleck.
Sunfleck frequency will be an additional factor for carbon gain during exposure to strong photon irradiance. Photosynthetic induction state diminishes to a minimum after 30 min in low light (see Figure 12.18 below) and some minutes are required to regain full capacity in strong light. Infrequent sunflecks are thus used with reduced efficiency.
Sunflecks and sun patches are of potential use to understorey plants for photosynthesis, but is this potential realised? Growth of understorey tree seedlings has been shown to be correlated with the amount of direct light received in sunflecks, and up to 60% of carbon gain in such environments has been attributed to this source. However, when compared with expected values based on the known steady-state response of plants to light, sunfleck utilisation is often below predicted values (Pfitsch and Pearcy 1989). Moreover, species vary in their capacity to utilise sunflecks. Watling et al. (1997a) measured the growth of four Australian rainforest species under simulated sunfleck regimes and showed that sunflecks contributed to growth in two species (Diploglottis diphyllostegia and Micromelum minutum), whereas the other two species (Alocasia macrorrhiza and Omalanthus novo-guinensis) were unable to make effective use of sunflecks.
Two components of a plant’s photosynthetic physiology will determine how the light in a sunfleck is used. Firstly, photosynthetic capacity (Pmax) will set a ceiling on the amount of light a plant can use. Secondly, a few minutes of illumination at least are needed for PCR cycle intermediates to reach critical levels, and this ‘induction requirement’ of photosynthesis determines how quickly a leaf can respond to an increase in photon irradiance.
Measured photosynthetic capacities of understorey plants are often low. When a leaf experiences a sunfleck, carbon fixation will increase to the point of light saturation. If an understorey plant could increase Pmax, it could utilise more light. But there are trade-offs. For example, higher respiration rates would increase the light-compensation point, and increase carbon losses during the low light periods separating sunflecks.
Regardless of photosynthetic capacity, the ability of a plant to use sunflecks is also affected by the induction requirement of photosynthesis. When a leaf that has been in low light for some time is exposed to an increase in photon irradiance, the rate of photosynthesis does not increase instantaneously to the new level. Instead, there is a gradual increase in assimilation which can take from 10–60 min for completion. This ‘induction period’ varies according to species as well as the induction state of the leaf concerned. Three different processes are involved; namely (1) buildup of PCR cycle intermediates and in particular ribulose-1,5-bisphosphate (RuBP), (2) light-dependent activation of Rubisco, and (3) light-dependent opening of stomata. Each of these processes follows a different time-course. Buildup of a metabolite pool is fastest (1–2 min), followed by Rubisco activation (2–5min) and finally stomatal opening (up to 60 min). Relaxation in low light is more protracted but generally occurs in the same sequence, leading to a decline in the induction state.
Thus, the longer a leaf has been in low light, the lower it’s the induction state. Figure 12.18 illustrates this for a leaf of Alocasia macrorrhiza, a plant common in rainforest understoreys and forest edges in eastern Australia. Fully-induced leaves were transferred to low light (10 µmol quanta m-2 s-1) for different lengths of time (up to 60 min). Their induction state was determined as the proportion of light-saturated photosynthetic capacity (Pmax) achieved within 2 min after return to saturating light. Induction loss in Alocasia, therefore, has a half time of about 25 min, but other species have been found to be either faster (e.g. Adenocaulon bicolor, an understorey herb from the redwood forests of western USA; Pfitsch and Pearcy 1989) or slower (e.g. Castanospora alphandii, a shade-tolerant tree from Australian rainforests; Watling et al. 1997a, b).
Chazdon and Pearcy (1986) showed that continuous light is not needed for induction to proceed. If leaves were subjected to a series of 60 s lightflecks (artificial sunflecks) separated by 2 min of low light, then induction state increased with each successive lightfleck. In nature, sunflecks are often clustered with a sequence of irregular bursts, separated by dull periods of variable duration. Under these circumstances, the state of induction might improve such that a plant will respond more rapidly to closing sunflecks in each sequence. This is supported by the data of Chazdon and Pearcy (1986), where the efficiency with which a sequence of lightflecks was utilised increased with successive lightflecks (efficiency was calculated as the actual amount of carbon fixed during a lightfleck relative to the amount predicted if there had been no induction period).
Carbon gain by fully induced leaves during lightflecks can exceed that expected, resulting in improved efficiency of light utilisation. This ‘intermittency phenomenon’ was noted by Kriedemann et al. (1973) in grapevine leaves exposed to high-frequency lightflecks. Similar investigations in a number of other species have also shown that such enhancement occurs only with short-duration lightflecks and is more prominent in fully induced leaves. Alocasia macrorrhiza showed improved efficiency of lightfleck utilisation by fully induced leaves only when lightflecks were of 40 s duration or less. Un-induced leaves needed lightflecks 10s or shorter (Chazdon and Pearcy 1986).
This apparent improvement in light use efficiency results from continued carbon fixation in low light (or darkness) following a lightfleck. During a short lightfleck, pools of triose phosphate and RuBP build up because carbon fixation runs transiently slower than the light reactions. This pool of PCR intermediates is then used for post-illumination CO2 fixation. In rainforest understoreys, where sunflecks are generally longer than a few seconds, this kind of enhancement is unlikely to be important. However, it may contribute significantly to carbon gain under crop canopies, where sunflecks are much shorter and more frequent.
One consequence of generally low photosynthetic capacities in understorey plants is a limited ability to process the light energy they absorb during strong sunflecks. This limited ability can also be exacerbated by a low induction state. Under these conditions, understorey plants will need to dissipate excess energy if they are to avoid photodamage. Field measurements of chlorophyll fluorescence from A. macrorrhiza show a decline in the quantum yield of photosynthesis (measured as Fv/Fm) during saturating sunflecks, indicating that photoprotective mechanisms are probably being engaged. Simultaneous assessment of the xanthophyll pigments shows that interconversion of violaxanthin to zeaxanthin is also occurring. After the sunfleck has passed, conversion of zeaxanthin to violaxanthin is extremely slow in species such as A. macrorrhiza, perhaps allowing a more rapid photoprotective response for subsequent sunflecks. However, quantum yield increases more rapidly than xanthophyll reconversion on return to low light, demonstrating a requirement for both high ΔpH and zeaxanthin for internal photoprotection to occur (Watling et al. 1997b).
Engagement of photoprotective mechanisms by shade-tolerant plants in an understorey environment may seem surprising, but serves to illustrate the extent of spatial heterogeneity in resource availability which is a feature of most habitats.