Barry Osmond
Plants often harvest more light than they can use in photosynthesis. When they are exposed to excess light there is an ever-present possibility of photoinhibition. This may happen when tree fall produces a rainforest gap and suddenly exposes seedlings adapted to life on a forest floor to sustained 10- or 20-fold increases in photon irradiance, or when water stress or low temperature restricts access to CO2 in sun plants. The photochemical efficiency of light utilisation (\(\phi_{PSII}\)) declines rapidly, and in most cases reversibly. Usually the excess light is wasted as heat, instead of being used to drive assimilation. These reversible changes in efficiency are known as photoprotection, and, if adequate, photoinhibitory damage to the water splitting PSII reaction center (photoinactivation) is avoided. Plants must constantly manage the trade-off between photoprotection and photoinactivation, while optimizing the photochemical efficiency of PSII.Some of the earliest systematic studies of photoinhibition were done by A. Ewart (1896) in Pfeffer’s laboratory in Leipzig 120 years ago (Figure 2). He examined the effects of excess light on the ability of chloroplasts in leaves to evolve O2. He detected this by examining the movement of O2-requiring bacteria towards photosynthetically active cells in leaf sections. Ewart is best remembered because he went on to translate three volumes of Pfeffer’s famous textbook (The Physiology of Plants) into English, and later became the first Professor of Plant Physiology in Australia (University of Melbourne, 1904).
12.1-FE-Fig-2.png
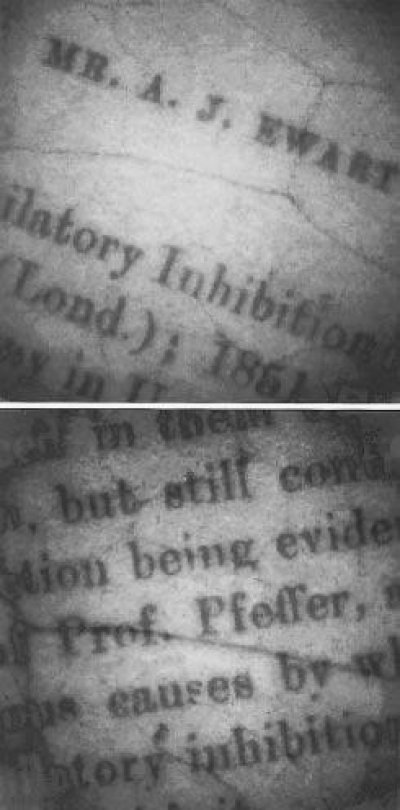
Figure 2 Photoinhibitory printing of excerpts from the first page of Ewart's paper on assimilatory inhibition on a leaf of the shade plant Cissus antarctica. A microfilm negative of the text was paper clipped to the leaf which was exposed to full sunlight for an hour. Chlorophyll fluorescence was subsequently imaged with a special video camera. Those areas of the leaf exposed to strong light under the text show severely reduced fluorescence due to photoinhibition. The latent image persists for several weeks because these shade plants repair photoinhibitory damage only slowly (Osmond et al. 1999)
Modern research on photoinhibition of light reactions of photosynthesis was strongly influenced by the Dutch biophysicist Bessel Kok (1956), responsible for so many advances in photosynthetic research, and by the early field studies of CO2 exchange by Björkman and Holmgren (1963) in Sweden. Björkman’s sabbatical in Australia in 1971 stimulated renewed interest in photoinhibition, and with Australian and German collaborators, research in his Stanford laboratory has repeatedly changed the way people think in this field (Powles 1984; Demmig-Adams and Adams 1992). Australian research in photoinhibition continues to attract attention (Anderson et al. 1997; Matsubara et al. 2012; Jia et al. 2013). Although modern research techniques such as in vivo chlorophyll fluorescence are much more quantitative and field-portable, the questions being probed are remarkably similar to those studied by Ewart!
By and large, leaves on most plants cannot avoid harvesting light, but some have evolved with external features to forestall absorption of excess radiation. For example, leaves of desert plants often reflect a large part of incident light, the high reflectivity being due to hairs, salt crusts (as in Australian saltbushes such as Atriplex nummularia) or epidermal waxes (Robinson et al. ). Such features are effectively mechanisms for external photoprotection. In others such as Townsville stylo (Macroptilium atropurpureum) leaves demonstrate very effective light-avoiding responses when water stressed. Ludlow and Björkman (1984) showed that if leaves of Townsville stylo were restrained perpendicular to incident sunlight, high-temperature-dependent photoinhibition ensued. It was obvious that unrestrained movement in the field preserved green and functional stylo leaves under conditions that accelerated senescence of leaves on adjacent herbs and grasses. By way of contrast, in some species like the compass plant (Lactuca scariola) leaves actually track the sun’s movement to maximise light interception.
The best defence against photoinhibition is a photosynthetic apparatus organised to take advantage of bright light. Sun plants have high capacities for CO2 fixation and retain high photosynthetic efficiency at relatively high photon irradiance (see Figure 12.9, and Case study 12.1). Depending on photosynthetic pathway and environmental conditions, sun plants may also sustain high rates of non-assimilatory electron transport in carbon recycling during oxygenase photorespiration (Section 2.3) and in the water-water cycle (Mehler reaction; Asada 1999) in the absence of net CO2 fixation during stress. Sun plants are also well endowed with photoprotective mechanisms that facilitate a reversible downregulation of PSII efficiency and stimulate wastage of absorbed photons as heat in the antennae pigment–protein complexes, before transfer to the reaction centre of PSII. These processes, linked to the energetic status of thykaloids and the interconversion of xanthophyll pigments (Section 12.1.2), provide internal photoprotection for PSII reaction centres (Demmig-Adams and Adams 1992). Sun exposed leaves of some epiphytes with low photosynthetic capacity, such as dodder and mistletoes have two xanthophyll cycles and so seem doubly photoprotected (Matsubara et al. 2002). Collectively known as mechanisms of non-photochemical quenching (NPQ) of chlorophyll fluorescence, these mechanisms that protect against photoinactivation have become a remarkably active realm of plant structural biology (Osmond 2015).
For the most part, these antennae-based processes seem to accommodate photon excess in most natural environments. However, when photon excess is sustained, especially in combination with other stresses, photoinhibitory damage leading to photoinactivation of PSII reaction centres may follow. The site of damage in most cases seems to be the psbA gene product, the D1 protein which is the most rapidly turned over protein in chloroplasts. The D1 protein is also the binding site for the triazine family of herbicides and accounts for their lethal effects. Turnover of D1 is accelerated in bright light, and it is often described as the ‘suicide protein’ (Aro et al. 1993; Chow and Aro 2005). Sun plants have high rates of chloroplast protein synthesis, and are thus able to repair damage to the critical D1 protein of PSII reaction centres more readily than in shade plants (Section 1.2).
Shade plants, in which the photosynthetic apparatus is organised to take advantage of low light, are poorly endowed with all of the above processes. Some, such as the archetypical Australian shade plants Alocasia and South American Tradescantia (Park et al. 1996) show the dynamic internal light avoidance property of chloroplast movement to anticlinal cell walls in strong light. However, when exposed to sustained bright sunlight, in excess of that encountered during growth, shade-tolerant plants such as Alocasia at the margins of Queensland rainforests suffer photoinhibitory damage. Structural organisation of the photosynthetic apparatus reflects these biophysical and bio-chemical realities at all levels, and not surprisingly the very different granal structures of shade and sun plants have important implications for photoinhibitory damage (Anderson and Aro 1994; Matsubara et al. 2012). In the short term, shade plants accommodate bright light in sunflecks without photoinhibitory damage, and even exploit it for additional post-illumination CO2 exchange (Chazdon and Pearcy 1986; Pearcy and Way 2012).
Somewhat surprisingly, the inner canopy shade leaves of some trees, especially those of tropical origin, are also equipped with two xanthophyll cycles (García-Plazaola et al. 2007). For example, inner canopy avocado shade leaves engage the near universal, initially rapidly relaxing violaxanthin cycle during a strong sun fleck. If these leaves are exposed to prolonged sunlight (following a cyclone for example) the slowly relaxing lutein epoxide cycle “locks in” photoprotection. A traffic-light inspired holistic summary light intensity- and time-dependent interaction of these photoprotective processes is illustrated in Fig. 3 (Jia et al 2013). Photoacclimation to bright light, the successful long-term accommodation of photoinhibitory processes, is genetically limited in many species. Yet in avocado it is manifest as the retrofitting of old shade leaves to perform as sun leaves, with increased photosynthetic capacity and enhanced photoprotection involving two xanthophyll cycles.
12.1-FE-Fig-3.png
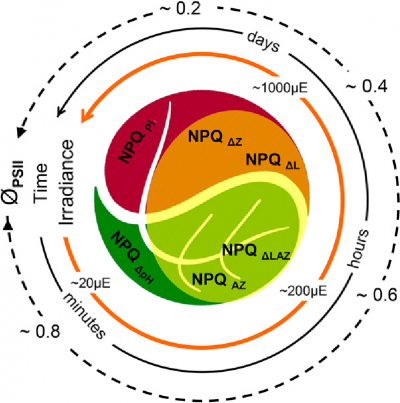
Figure 3 The Yin and Yang of photoinhibition. Depending on genotype, irradiance and time the extent of photoinhibition (indicated by decline in ϕPSII) proceeds through engagement of internal mechanisms of photoprotection (indicated by the extent of NPQ). Rapidly reversible (seconds to minutes) NPQΔpH potentiates the more slowly reversible (minutes to hours) de-epoxidation of violaxanthin to antheraxanthin and zeaxanthin (NPQΔAZ) in light harvesting antennae of PSII in thylakoid membranes. In some plants, such as avocado, this photoprotection is augmented by a second de-epoxidation of lutein epoxide to lutein (NPQΔLAZ) that may persist for days. If still inadequate it will be followed by similarly slowly recoverable PSII reaction centre photoinactivation (NPQPI). (Reproduced from Jia et al. 2013. Plant Physiology 161, 836-852; Copyright American Society of Plant Biologists)
Although unicellular algae such as Chlamydomonas sp. have been widely used to research mechanisms of photoinhibitory damage (Förster et al. 2005), relatively much less is known of photoinhibition in either marine or freshwater environments. Under natural conditions, vertical movement of unicellular algae in water columns is an important determinant of photon exposure and photoinhibitory responses, which involve many of the same processes as in higher plants (Franklin et al. 2003). In addition, the ubiquitous marine macrophyte Ulva is susceptible to desiccation and high-temperature-dependent photoinhibitory damage in rock pools and estuaries when low tides occur at midday. The diversity of photosynthetic pigments among marine macrophytic algae suggests several alternative photoinhibitory mechanisms that are currently under investigation.
Clearly, photoinhibition is an integral and indispensable component of photosynthesis. The inefficiencies it produces in light utilisation are essential to the stability of the photosynthetic apparatus in organisms that depend on light for life, and especially in environments where they can do little to regulate the incoming flux of this basic resource. The costs of these inefficiencies remain difficult to estimate and the extent to which plant distribution in relation to sunlight is governed by photoinhibitory responses, remains controversial. Perhaps one of the most convincing examples is the interaction of bright light and low temperature which restricts re-establishment of eucalyptus seedlings to the shaded south side of parent trees on the Southern Highlands of New South Wales (Ball et al. 1991; Case study 14.1). New techniques for the remote sensing of chlorophyll fluorescence that monitor photosynthesis, photoprotection and photoinhibition offer exciting insights that will facilitate integration of these processes from leaves to canopies (Nichol et al. 2012) with the added prospect of ground truth for satellite observation of solar induced fluorescence at the landscape level. As with most aspects of plant biology today, genetic manipulations of many aspects of the above component photoprotective mechanisms are mooted to benefit plant productivity through mitigation of various aspects of photoinhibition (Ort et al. 2015).
This revision and update of Feature Essay 12.1 is dedicated to the memory of Professor Jan M Anderson (1932-2015) for her outstanding leadership and encouragement of Australian research in photosynthesis.
References
Anderson JM, Aro E-M (1994) Grana stacking and protection of photosystem II in thylakoid membranes of higher plant leaves under sustained high irradiance: an hypothesis. Photosyn Res 41: 315–326.
Anderson JM, Park Y-I, Chow WS (1997) Photoinhibition and photoprotection in nature. Physiol Plant 100: 214–223.
Aro E-M, Virgin I., Andersson B (1993) Photoinhibition of photosystem II. Inactivation, protein damage and turnover. Biochim Biophys Acta 1134, 113–134.
Asada K (1999) The water-water cycle in chloroplasts: scavenging of active oxygens and dissipation of excess photons. Annu Rev Plant Physiol Plant Mol Biol 50, 601-639.
Ball MC, Hodges VS, Laughlin GP (1991) Cold-induced photoinhibition limits regeneration of snow gum at treeline. Funct Ecol 5: 663–668.
Björkman O, Holmgren P (1963) Adaptability of the photosynthetic apparatus to light intensity in ecotypes from exposed and shaded habitats. Physiol Plant 16, 889–914.
Chazdon RL, Pearcy RW (1986) Photosynthetic responses to light variation in rainforest species. II Carbon gain and photosynthetic efficiency during lightflecks. Oecologia 69: 524–531.
Chow WS, Aro E-M (2005) Photoinactivation and mechanisms of recovery. In ‘Photosystem II. The light-driven water: plastoquinone oxidoreductase advances in photosynthesis and respiration’, Vol. eds TJ Wydrzynski, K Satoh, JA Freeman. 627–648, Springer, Dordrecht, The Netherlands)
Demmig-Adams B, Adams III WW (1992) Photoprotection and other responses of plants to high light stress. Annu Rev Plant Physiol Plant Mol Biol 43: 599–626.
Ewart AJ (1896) On assimilatory inhibition in plants. J Linnean Soc 31: 364-461.
Förster B, Osmond CB, Pogson BJ (2005) Improved survival of very high light and oxidative stress is conferred by spontaneous gain-of-function mutations in Chlamydomonas. Biochim Biophys Acta (Bioenerg) 1709, 45-57.
Franklin LA, Osmond CB, Larkum AWD (2003) Photoinhibition, UV-B and algal photosynthesis. In Photosynthesis in Algae. Advances in Photosynthesis and Respiration, Vol. eds AWD Larkum, SE Douglas, J A Raven. 351-384, Kluwer, Dordrecht.
García-Plazoala J-I, Matsubara S, Osmond CB (2007) The lutein epoxide cycle in higher plants: its relationship to other xanthophyll cycles and possible functions. Funct Plant Biol 34, 754-779.
Jia HS, Förster B, Chow WS et al. (2013) Decreased photochemical efficiency of Photosystem II following sunlight exposure of shade-grown leaves of avocado (Persea americana Mill.): because of, or in spite of, two kinetically distinct xanthophyll cycles? Plant Physiol 161: 836-852.
Kok B (1956) On the inhibition of photosynthesis by intense light. Biochim Biophys Acta 21: 234–244.
Ludlow MM, Björkman O (1984. Paraheliotropic leaf movement in Siratro as a protective mechanism against drought-induced damage to primary photosynthetic reactions: damage by excessive light and heat. Planta 161: 505–518.
Matsubara S, Gilmore AM, Ball MC et al. (2002) Sustained down regulation of photosystem II in mistletoes during winter depression of photosynthesis. Funct Plant Biol 29: 1157-1169.
Matsubara S, Förster B, Waterman M et al. (2012) From ecophysiology to phenomics: some implications of photoprotection and shade-sun acclimation in situ for dynamics of thylakoids in vitro. Phil Trans Royal Soc London B 367: 3503-3514.
Nichol CJ, Pieruschka R, Takayama K et al. (2012) Canopy conundrums: building on the Biosphere 2 experience to scale measurements of inner and outer canopy photoprotection from the leaf to the landscape. Funct Plant Biol 39: 1-24.
Ort D R et al. (2015) Redesigning photosynthesis to sustainably meet global food and bioenergy demand. Proc Nat Acad Sci USA 112: 8529-8536.
Osmond B, Schwartz O, Gunning B (1999) Photoinhibitory printing on leaves, visualised by chlorophyll fluorescence imaging and confocal microscopy, is due to diminished fluorescence from grana. Aust J Plant Physiol 26: 717-724.
Osmond B (2015) An ecophysiologist’s apology. Book Review: Non-photochemical Quenching and Energy Dissipation in Plants, Algae and Cyanobacteria, Advances in Photosynthesis and Respiration, Vol 40. eds B. Demmig-Adams, G. Garab, W.W. Adams III and Govindjee (eds) (2014), Springer, Dordrecht. Photosyn Res 124:127-130
Park Y I, Chow WS, Anderson JM (1996) Chloroplast movement in the shade plant Tradescantia albiflora helps protect photosystem II against light stress. Plant Physiol 111: 867-875.
Pearcy RW, Way DA (2012) Two decades of sunfleck research: looking back to move forward. Tree Physiol 32 1059-1061.
Powles SB (1984) Photoinhibition of photosynthesis by visible light. Annu Rev Plant Physiol 35: 15-44.
Robinson SA, Lovelock CE, Osmond CB (1993) Wax as a mechanism for protection against photoinhibition - a study of Cotyledon orbiculata. Bot Acta 106: 307-312.