Fig14.12.png
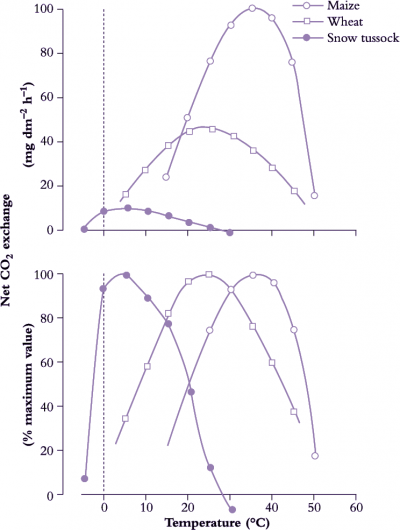
Figure 14.12. Plant species show characteristic variation in the way photosynthetic tissue responds to temperature. Maize (a subtropical C4 species) has a high maximum rate of CO2 assimilation with a high optimum temperature, while wheat (a temperate C3 species) has a lower maximum rate and a lower optimum temperature. (a) Absolute values; (b) shows those same data normalised to 100% at optimum temperature. Alpine plants (C3 species) have an even lower optimum temperature and may show CO2 assimilation below 0 °C, but their maximum rates of photosynthesis are often low compared with warmer climate species. Based on I F Wardlaw (1979).
CO2 assimilation underpins plant productivity and is therefore central to any analysis of the response of plants to a change in temperature. In photosynthetic terms, plants can be divided broadly into the groups discussed earlier.
Some C3 species such as snow tussocks have an optimum temperature for CO2 assimilation as low as 5°C, but it is important to note that absolute rates at this temperature may be relatively low (Figure 14.12). Most temperate grasses and cereals as well as many woody species have temperature optima in the range from 15°C to 25°C and within this range many C3 species show only small changes in CO2 uptake. In contrast to temperate C3 species, CO2 assimilation by C4 species increases considerably with a rise in temperature from 15°C to 30°C and optimum temperatures may be greater than this (Figure 14.12). Rice, a subtropical C3 species, has a higher temperature optimum for CO2 assimilation than temperate C3 species. Under high light, C4 species have a characteristically greater photosynthetic rate than the C3 species, but these differences disappear and may be reversed at low temperature. Growth temperatures may also influence the optimum temperature for net photosynthesis, which may therefore vary with season or location. However, modifications leading to an improvement in photosynthesis at high temperatures can result in decreased performance at low temperatures and vice versa.
A combination of at least two factors may be associated with the failure of C3 species to respond more favourably to high temperature in terms of CO2 assimilation. One is the limit placed on photosynthesis by ambient CO2 (Figure 14.13), and a second is the concurrent rise with increasing temperature of light-stimulated photorespiration, which is effectively absent from C4 species. Increased atmospheric CO2 inhibits photo-respiration and results in a much greater uptake of CO2 in response to increased temperature in C3 species.
Fig14.13.png
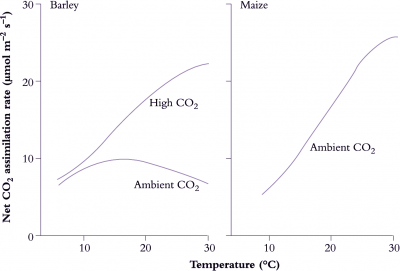
Figure 14.13. Maize (a subtropical C4 species) has a high optimum temperature (~30 °C) for CO2 assimilation, while barley (a temperate C3 species) has a lower, but less distinct optimum (~15 °C). Doubling CO2 has little effect on CO2 assimilation rate by maize (not shown here), but greatly increases the absolute rate and optimum temperature for barley by suppressing photorespiration. This O2-dependent loss of carbon increases with temperature and is largely responsible for the low optimum temperature of photosynthesis in many C3 species. Based on Labate, Adcock and Leegood (1990) Planta 188, 547-544.
While net photosynthesis is the resulting balance between gross photosynthesis and respiration, low or even negative net photosynthetic rates can have a significant effect on productivity. An example of this would be photosynthesis by the green pod of many legumes where the net uptake of CO2 is low because of a high rate of pod and seed respiration. A rise in temperature will result in greater respiratory losses from the pod and a reduction in net uptake of CO2, but this does not diminish the importance of pod photosynthesis.
Variation in stomatal resistance could be another factor associated with temperature effects on net assimilation of CO2. Adaptation to high temperature can be related to photosystem II electron transfer, the stability of chloroplast membrane-bound enzyme activities and the stability of the photosynthetic carbon metabolism enzymes that require light for activation. For example, enhanced assimilation of CO2 by rice at high temperature, in comparison with the more temperate C3 species, is associated with a greater response of ribulose-1,5-bisphosphate carboxylase in rice to increasing temperature.
In chilling-sensitive species such as maize, sorghum and mung bean, chlorophyll formation and chlorophyll destruction both occur in light at low temperatures. However, once greening has occurred quite low temperatures (but not usually freezing) can be tolerated as long as these occur during darkness. Low-temperature tolerance is also associated with a high level of strategic enzymes such as Rubisco, protein stability and membrane lipid composition.
Consideration must also be given to possible indirect effects of temperature on photosynthesis. For example, a change in root growth due to a change in temperature could alter the supply of nutrients or growth regulators, such as cytokinins, to the shoots. It is common to find a build up of non-structural carbohydrates in many parts of a plant under low-temperature conditions, a response indicating that growth is more sensitive to low temperature than photosynthesis. However, feedback inhibition of photosynthesis associated with this excess carbohydrate accumulation under low-temperature conditions occurs in a number of species. In summary, it is important to take into account the possibility of indirect effects of temperature on photosynthetic tissue when looking for genetic differences in photosynthetic responses to temperature.