The much slower diffusion of gases in water, compared with in air (Table 18.2), presents a challenge to plants that become submerged.
Gas exchange of submerged plants is greatly impeded by their environment. Although the distances across diffusive boundary layers (DBL) around leaves are the same order of magnitude in water and air, the 10,000-times slower diffusion in water results in the high resistance to gas exchange across the DBL in water. Consequently, submerged aquatic plants have developed adaptive features of their leaves that reduce the DBL, to facilitate exchange of O2 and CO2 with the surrounding water (Table 18.3).
Fig_18.3.png
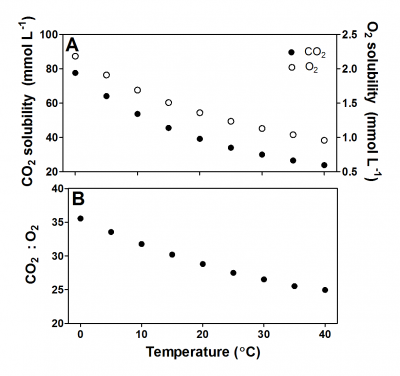
Figure 18.3. Solubility of pure CO2 and O2 in water (A) and the ratio of CO2:O2 solubility versus temperature (B). The solubility of CO2 at 20 °C is ~ 29-fold higher than the solubility of O2, and when temperature rises the solubility of CO2 decreases faster than for O2. Data for O2 from Himmelblau and Arends (1959); for CO2 from Wilhelm, Battino and Wilcock (1977).
Other leaf features/properties can also differ between terrestrial wetland plants and submerged aquatic plants, such as: venation, lignification, stiffness, surface topography, differences between adaxial and abaxial surfaces, and in the case of some halophytic wetland species presence of salt bladders and glands (Table 18.3).
In addition to the slow diffusion, the solubility of O2 in water is poor. One litre of air contains approximately 33-fold more O2 than one litre of water at 20 °C at sea level (101 kPa). Temperature affects the solubility of O2; the solubility decreases with increasing temperature (Figure 18.3). Imagine a kettle that heats up; the water starts bubbling long before it reaches the boiling point because the solubility of gaseous N2 and O2 steeply decreases as the temperature rises.
Salinity also affects the solubility of O2 in water. Sea water contains 35 ppt (parts per thousand) salt, which is approximately 550 mM NaCl, and at 20 °C contains only ~231 µmol O2 L-1 as compared to freshwater that holds ~290 µmol O2 L-1.
Like for O2, the solubility of CO2 also decreases with increasing temperature and salinity. However, the chemistry of CO2 in water is more complicated than for O2, as CO2 reacts with water in the following pH-dependent equilibria (Figure 18.3 and its caption). CO2 reacts with water (H2O) and forms carbonic acid (H2CO3). However, H2CO3 dissociates immediately into a proton (H+) and bicarbonate (HCO3-) so the dissolution of CO2 into water causes pH to drop. At high pH, HCO3- can further dissociate into a second H+ and carbonate (CO32-). The sum of CO2, (H2CO3), HCO3- and CO32- is referred to as dissolved inorganic carbon (DIC) and the relative distribution of the three main forms of inorganic carbon is determined by pH (Figure 18.4).
Fig_18.4.png
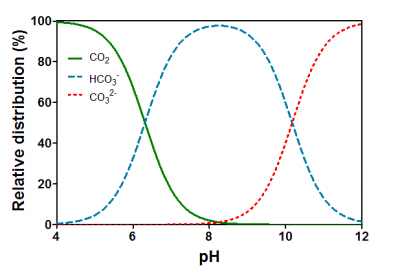
Figure 18.4. Relative distributions of CO2, HCO3- and CO32- as a function of pH in water. When CO2 dissolves in water, it reacts with water: CO2 + H2O ↔ H2CO3 ↔ HCO3- + H+ ↔ CO32- + H+. The apparent pKa1 is 6.532; only a little CO2 is converted into carbonic acid while the majority remains as CO2(aq). pKa2 is 10.329. So, water pH influences the availability of CO2 to submerged plants. Below pH 6 most of the dissolved inorganic carbon is available as CO2. Between pH 7 and 10, the alkaline ion HCO3- dominates, which can be used only by the most specialised aquatic plants as an inorganic carbon source. Only at pH higher than 10, a significant proportion of the dissolved inorganic carbon is in the form of CO32-, which plants cannot use in photosynthesis. Stumm and Morgan (1996).
The concentrations of O2 and CO2 can differ markedly between water bodies. In net heterotrophic systems the waters contain dissolved CO2 concentrations above that when in equilibrium with air; respiration by organisms consuming labile carbon compounds results in depletion of O2 but enrichment of CO2. By contrast, in net autotrophic systems, photosynthesis exceeds respiration and so depletes CO2 and elevates O2 in these waters. So, O2 concentrations in floodwaters can range from severely hypoxic (net heterotrophic) to above air equilibrium (net autotrophic); and both these conditions can occur in diel patterns (respiration only during night; photosynthesis dominates during day), for example as measured in fields of submerged rice.
Besides the slow diffusion of CO2 in water, photosynthesis by submerged plants can also be limited by light. In water, light attenuation follows an exponential function,
\[I=I_0 \text{e}^{-z\epsilon}\]
where I is the available light at a given depth (z), I0 is the light level at the surface, ε is the extinction coefficient, and e is the exponent. The extinction coefficient of water itself is 0.03 m-1 so in ultra clear water, rooted plants could grow down to a depth of 75 m where 10% of the surface light would still be present, which happens to be the approximate depth limit of seagrasses). However, dissolved coloured organic carbon (e.g. humic acids), chlorophyll in planktonic algae, and particles suspended in the water (e.g. silt), all reduce light penetration in floodwaters. In turbid floodwaters, light attenuation can be as much as 90% in the upper 10 cm, whereas more typical depths for a 90% reduction of light in floodwaters might be 0.5 - 2 m.