3.0-Ch-Fig-3.12.jpg
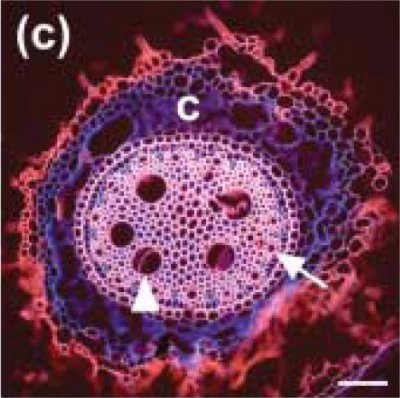
Figure 3.12 Cross section from barley root grown in soil; coleoptile node axile root, bar 100 µm. C is cortex, arrow points to peripheral xylem and arrowhead points to inner xylem. Extensions from the epidermis (red) are root hairs. Section was stained with rhodamine B and viewed with UV fluorescence optics. Micrograph, M. Watt. (Reproduced from New Phytol 178: 135-146, 2008)
The diameter of xylem vessels can be as small as 10 µm as in Arabidopsis, 60-100 µm in the roots of wheat and rapid growing annuals like maize, to over 100 µm in trees. Remembering that trees can be over 100 m in height, the conductive efficiency of xylem conduits is essentials for plants to move water to the canopy at rates that satisfy the transpirational water loss at the leaf surface. These dimensions are for the vessels with maximum diameter, the late metaxylem in the central part of the stele (e.g. Figure 3.12).
The diameter of xylem vessels in a given species varies greatly with root type (Watt et al. 2008). For example, in wheat and barley, the diameter varies from 10 to 60 µm depending on position within the stele (central or peripheral), and the type of root (seminal or nodal). Figure 3.12 shows a section of a nodal root from barley.
A wider xylem diameter translates to an increase in conductive efficiency that can be appreciated by revisiting equation (6). From the Hagen-Poiseuille Law, which shows that flow increases with the fourth power of the radius, we can see that a four-fold increase in the radius of a tube leads to a 256 fold increase in the volumetric flow rate.
In addition to allowing for high rates of water flow, the xylem must also protect the plant against formation and spread of gas bubbles. For xylem sap to sustain tensions required in tall trees, there must be no gas bubbles in the system. Cohesion breaks down if there is a single ‘nucleation site’ on which bubbles can form and enlarge. On the other hand, sap normally contains dissolved gases which, surprisingly, do not disrupt the system provided there are no nucleation sites available. Even the rigid walls of xylem vessels are compatible with high xylem tensions, attracting water by adhesion, which is essential for transport.
Surface tension acts as an interfacial water–air stopper, preventing air from being sucked into the many millions of tiny pores present in all plant cell walls. For example, water delivered to leaf cells by xylem vessels passes through these tiny menisci, which act effectively as non-return valves, so preventing air from being sucked into the xylem (Section 3.3). Surface tension also explains how water in leaves remains under strain within an essentially porous system through which water flows.
3.0-Ch-Fig-3.13.jpg
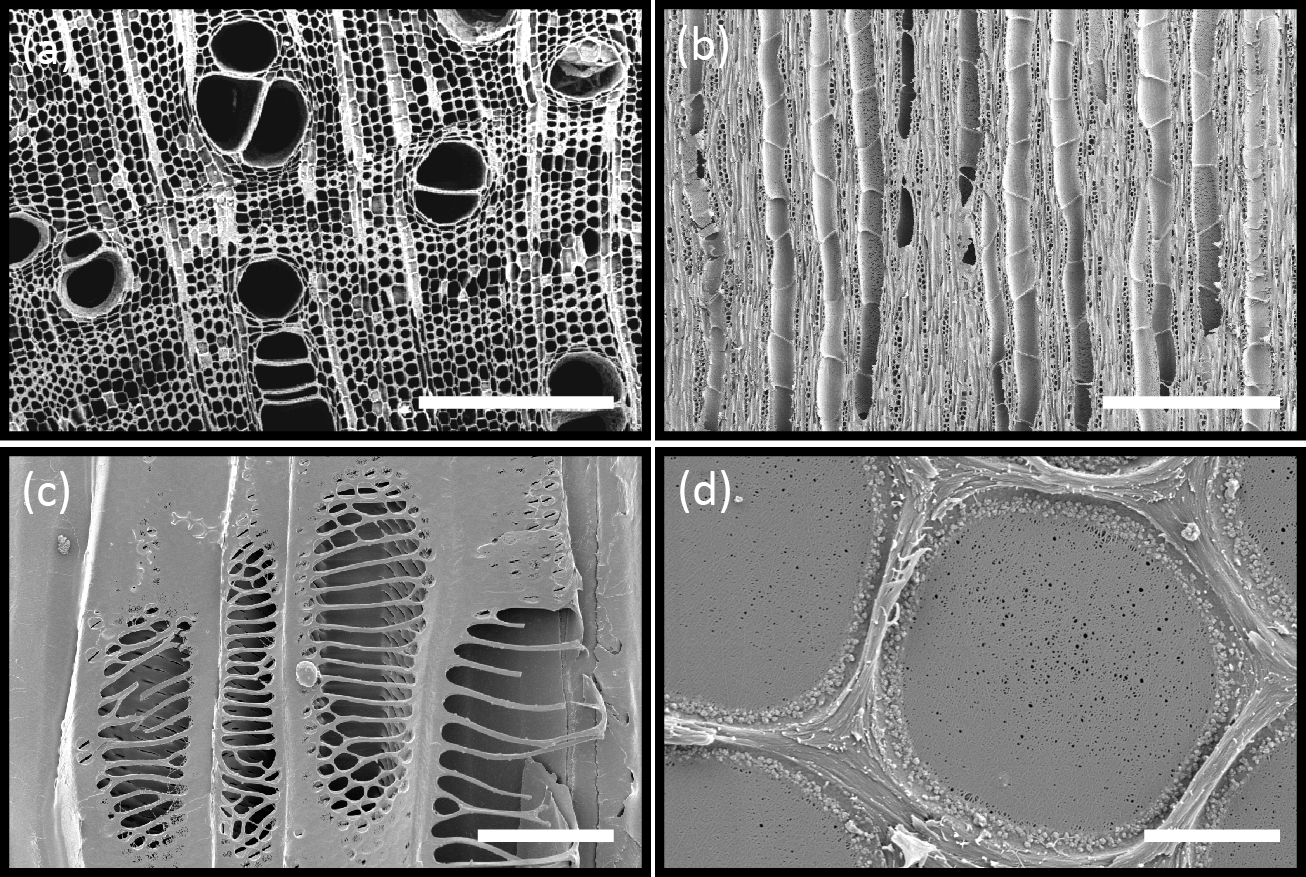
Figure 3.13 (a) Scanning electron micrograph (SEM) shows a transverse section of xylem tissue in Brachychiton australis. Large xylem vessels are surrounded by fibres and parenchyma; scale bar, 500 μm. Xylem vessels are dead at maturity and form long hollow tubes that minimise the resistance to water flow through the plant. Connecting intervessel walls contain bordered pits, cavities in the lignified secondary cell walls that allow for transfer of water between vessels. (b) Longitudinal section showing vessels in the xylem tissue of Fraxinus americana. Vessels are made up of repeated individual units (vessel elements) that are joined end to end by perforation plates; scale bar, 400 μm (c) SEM of the finely sculptured scalariform perforation plates in Betula ermanii xylem. Water passes easily from one xylem vessel to another by this route; scale bar, 20 μm (d) SEM showing surface view of the pit membrane with secondary wall removed by sectioning; scale bar, 2 μm. Tiny pores allow the movement of water between vessels but limit the movement of gas and pathogens. Bordered pits act as the safety valves of the plant hydraulic system. (Images courtesy B. Choat and S. Jansen).
Vascular transport systems have evolved to become amazingly reliable despite the metastable condition of the sap (existing as a liquid below its vapour pressure). From primitive, thickened, hollow cells, increasing specialisation has produced greater elongation and thickening of the tubes (Figure 3.13a,b). Xylem walls contain pits, in which zones of the primary wall known as ‘pit membranes’ allow water to be transmitted between vessels efficiently, while preventing a gas phase spreading through the interconnected system of vessels and blocking transport through embolisation (the blockage of a fluid channel with a bubble of gas) (Figure 3.13d). No living membrane is present in these wall structures. The efficiency with which pit membranes isolate adjacent vessels is shown in Figure 3.11b (in the previous section) where mercury, a highly cohesive liquid, is drawn into specialised bordered pits of pine tracheids without being able to exit into neighbouring tracheids.
Vascular systems have evolved from plant species possessing only fibres and tracheids, for example the more primitive Tasmannia, to more advanced plants possessing vessels which resemble the unicellular tracheids in structure but are much wider and longer and originate from a number of cell initials fused together. Lignin thickening patterns have also evolved. Some thickening designs, such as annular and spiral, allow the tubes to extend longitudinally while supplying growing organs.
When elongation growth has ceased, an organ can be provided with more efficient pipes of larger bore and with stronger thickenings, in reticulate and scalariform patterns (Figure 3.13c). Pit fields which allow water transport across vessel walls can also be simple, unreinforced structures (simple pits) or more elaborate bordered pits in which secondary cell walls mechanically support the pit membrane. All these forms of pits can prevent air in an air-filled conduit from spreading to adjacent conduits which are conducting water under strong suction. Reinforcement of the walls around pits allows pit membranes to be as large as possible and thereby maximise water exchange between vessels.